The Role of Tumor Suppressor Gene Epigenetic Regulation in the Prognosis of Chemotherapy Resistance and Aggressiveness of Breast and Lung Cancer
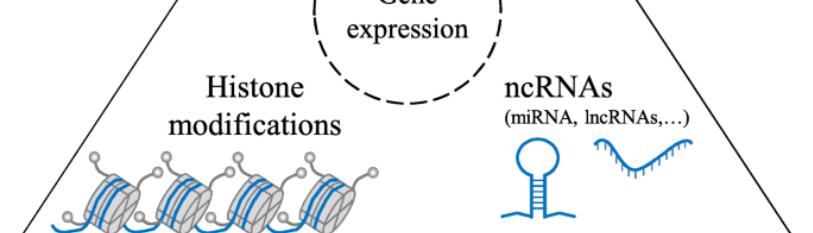
Navrinder Kaur1*, Aditi Saini2, Arnav Walia3, Rajesh Saxena2, Poorvee Mathur4 and Vinitaa Jha5
1*Max Society of Medical Academics Innovation and Research, Max healthcare Institute Ltd., Saket, Delhi, 110017
2Max Healthcare, Office of Clinical Research, 2, Press Enclave Road, Saket, New Delhi, India, 110017
3Dr. Shroff’s Charity Eye Hospital, Kedarnath Lane, Daryaganj, Delhi, 110002
4Max Healthcare, 2, Press Enclave Road, Saket, New Delhi, India, 110017
5Clinical Directorate, Max Healthcare Institute Ltd., Sector 59, Gurgaon, Haryana, 120001
*Corresponding author: Navrinder Kaur, Max Society of Medical Academics Innovation and Research, Max healthcare Institute Ltd., Saket, Delhi, 110017
Citation: Kaur N, Saini A, Walia A, Saxena R, Mathur P, Jha V. (2023) The Role of Tumor Suppressor Gene Epigenetic Regulation in the Prognosis of Chemotherapy Resistance and Aggressiveness of Breast and Lung Cancer. Adv Clin Med Res. 4(3):1-17.
Received: July 7, 2023 | Published: July 31, 2023
Copyright© 2023 genesis pub by Kaur N, et al. CC BY-NC-ND 4.0 DEED. This is an open-access article distributed under the terms of the Creative Commons Attribution-Non Commercial-No Derivatives 4.0 International License., This allows others distribute, remix, tweak, and build upon the work, even commercially, as long as they credit the authors for the original creation.
DOI: https://doi.org/10.52793/ACMR.2023.4(3)-58
Abstract
DNA repair gene regulation is realized with the participation of epigenetic mechanisms those are epigenetic in nature and may include chromatin modification (DNA CpG islands with methylation and histone modifications), chromatin remodeling (shifting of chromatin basic unit, a nucleosome), regulation with non-coding RNAs (small noncoding RNAs also known as micro RNAs and long noncoding RNAs). Chromatin epigenetic modifications in DNA repair genes and corresponding modifying enzymes are studied with the aim to choose the optimal chemo- and targeted cancer therapies. Although, multiple chemotherapeutic compounds are not always introduced in medical practice, because the level of DNA methylation and the histone protein modification status (such as acetylation/deacetylation;methylation/demethylation;phosphorylation/dephosphorylation;ubiquitilation/deubiquitilation), as well as their significance need additional confirmation with the use of different experimental methodologies.
Therefore, it is worth to note that the process of DNA methylation and histone protein modifications are interdependent: from one hand, enzymes recognizing lysine methylation in histones could lead to DNA methylation and, from the other hand, DNA methylation could induce corresponding histone modifications in chromatin. Moreover, epigenetic modifications occurring during DNA repair gene expression and the level of participating enzymes could be predictive markers of breast and lung cancer chemotherapy resistance and prognostic markers of their aggressiveness.
In this review, the DNA and histone modifications, regulation with microRNAs and epigenetic changes during splicing process will be discussed in the following DNA repair genes: BRCA1, BRCA2, RAD51 (DNA double strand repair), ERCC1 (DNA single strand repair), MLH1 (miss match repair, MMR) in breast and lung cancer. The role of these modifications and participating enzymes as predictive markers of breast and lung cancer chemotherapy resistance and prognostic markers of their aggressiveness will be highlighted.
Keywords
DNA single strand repair; BRCA1 gene transcription; DNA methylation; DNA repair of BRCAs; Chemotherapy resistance
Introduction
It is already universally recognized for more than a decade that the development of cancer including the most common carcinomas deriving from epithelial cells (lung, breast cancer, etc.) is associated not only with the genetic changes such as mutations but also with the aberrant nature of epigenetic regulation of the gene expression [1,2]. Dysregulation of gene expression epigenetic control could occur at the level of both transcription initiation at the promoter/enhancer regions and alternative splicing at the intragenic sites. In these cases, abnormal DNA methylation, histone modification/remodeling and regulation with the non-coding RNAs (microRNAs and long non coding RNAs also known as lncRNAs) could progress to the stage of cancer.
It is commonly cited that three major nuclear processes, DNA repair, transcription and splicing, are realized in the context of chromatin. Therefore, the epigenetic regulation with the involvement of multiple chromatins modifying enzymes is the characteristic feature of these processes [3]. The most basic unit of chromatin, is nucleosome, and it consists of a DNA segment wrapped around the histone octamer. This histone octamer is composed, of two pairs of histones i.e., H3/H4 and two another pairs of histones H2A/H2B. To link the and stabilize the structure of histone particle H1 linker histone stays responsible. The scheme of nucleosome formation is presented on (Figure 1) [4-6].
Figure 1: Chromatin structure formation. Histones H3 and H4 exist as dimers in the cytoplasm and in the nucleus before the incorporation into the chromatin. Then, histone H3/H4 dimers incorporate in DNA followed with the association of histone H2A/H2B dimers. The nucleosome formation is terminated at the addition of linker histone H1.
In many cases the epigenetic mechanism is mostly involved in the regulation of the DNA repair gene expression, especially of BRCA1 gene [7-10]. There are several types of DNA repair such as, DNA double strand break repair via the homologous recombination with the participation of BRCA1, BRCA2, RAD51 [11], DNA single strand break repair involving ERCC1 [12], miss match repair (MMR) with the participation of MLH1 [13].
The epigenetic regulation of the DNA repair gene expression is mostly associated with long-term processes. For example, at hypoxia, which is one of most important hallmarks of the cancer disease, there are several levels of gene expression regulation (Fig. 2). At the acute hypoxic stress that is realized in minutes, posttranslational modifications of DNA repair proteins regulate their activity. Further, if hypoxia condition persists for hours and days, the process of regulation of DNA repair gene expression takes place at the transcription and translation phase. Prolonged moderate, in other words, chronic hypoxia lasting for days and weeks induces epigenetic modifications in DNA repair genes (Figure 2).
Figure 2: DNA repair gene expression regulation at hypoxia. Different stages ofDNA repair gene expression regulation at hypoxia are presented. Firstly, during several minutes of the acute hypoxic stress the posttranslational modifications of DNA repair proteins regulate their activity. Secondly, at hypoxia conditions persisting for hours and days the regulation of DNA repair gene expression takes place at the level of transcription and translation. Thirdly, prolonged moderate hypoxia lasting for days and weeks induces epigenetic modifications in DNA repair genes.
Several DNA repair genes were investigated with the aim to identify particular epigenetic modifications characteristic for certain cellular processes. As shown in Figure 3, in hypoxia conditions at the BRCA1 DNA double strand break repair gene promoter the deacetylation of lysines (Lys/K) in chromatin histone protein, namely in H3K9ac, takes place followed with the methylation of H3K9. Also, the demethylation of H3K4me occurs in the BRCA1 gene promoter. Taken together, these events lead to the inhibition of BRCA1 gene transcription (Figure 3A is based on the results obtained of the study [14]). The (EMT) epithelial mesenchymal transition is characterized with the loss of H3K4me methylation at the BRCA1 gene promoter which resultantly do inhibition of BRCA1 gene transcription as well (Figure 3 is based on the results obtained in a study [15] (Figure 3).
Figure 3: Epigenetic regulation of BRCA1 gene expression at the hypoxia and at the epithelial-mesenchymal transition (EMT). (A)Histone modifications in BRCA1 gene promoter in hypoxia conditions. Histone methyltransferase specific for Н3K9 methylates Lys9 (K9) in histone Н3 that was preliminary deacetylated. In addition, histone demethylase specific for Н3K4me demethylates Lys4me (K4me) also in histone Н3. The combination of these modifications leads to the inhibition of BRCA1 gene transcription. (B) Histone modifications in BRCA1 gene promoter at the EMT.Histone demethylase specific for Н3K4me2/3 demethylates Lys4me2/3 (K4me2/3) in histone Н3 at BRCA1 gene promoter at the activation of Wnt signaling pathway involved in the EMT.
Besides DNA repair, there are at least two steps in gene expression regulation that are dependent on epigenetic mechanisms: transcription activation/initiation and alternative splicing, the latter generating the diversity of mRNA species from the single gene followed with the synthesis of corresponding protein isoforms. Recently multiple mechanisms are involved in the epigenetic regulation of alternative splicing events were revealed [16-17]. These mechanisms involve the activity of several histone modifying enzymes leading to the formation of modified chromatin regions containing H3K4me3 [18], H3K9me3 in complex with the heterochromatin protein HP1γ [19], H3K36me3 [20], H3K27me3 [21], as well as acetylated histones [22]. It was also shown that the BRM subunit of the chromatin remodeling complex SWI/SNF [23] and the components of RNA interference machinery, Argonaute 1 and 2 proteins (AGO1, AGO2) [24], participate in the regulation of splicing. All together, these processes tend to decrease the elongation rate of the RNA polymerase II (RNA pol II) due to the generation of the interfering chromatin structures, therefore facilitating the inclusion of alternative exons (Figure 4).
Figure 4: miRNAs involved in breast and lung cancer development and progression. Several miRNAs with partially overlapped pattern participate in breast and lung cancer development and progression. They are also used as biomarkers of these types of cancer. Detailed description of their functions is presented in the text.
Recently, a lot of data accumulated indicating that there is a crosstalk happen between the DNA and histone protein epigenetic modifications [25]. Particularly, it concerns the methylation of the CpG islands in chromatin DNA and the methylation of K in chromatin histone proteins: the enzymes catalyze the DNA methylation as a consequence of K histone methylation recognition, and, in the reverse situation, the DNA methylation status could induce the histone modifications in the chromatin with the participation of corresponding enzymes [26]. It is important to note that many chromatin modifying enzymes such as K-specific demethylases and deacetylases as well as bromodomain containing proteins recognizing acetylated K amino acid residues of histones in certain chromatin regions and several other chromatin modifying and recognizing enzymes represent targets for anti-cancer therapy [27,28]. In this regard, it is worth to mention the intercalating substances that interfere with the binding of reader bromodomains containing proteins, such as BET family chromatin adaptors, to the acetylated K residues in chromatin histones. As a result, intercalating substances inhibit transcriptional activation. Classical example is treatment of the cancers induced by the MYC expression activation, such as Burkitt lymphoma and acute myeloid leukemia, with the intercalating compound (+)-JQ1 that inhibit MYC gene transcription through the displacement of BET family chromatin adaptors from MYC gene promoter [29]. Therefore, a knowledgebase called ChEpiMod was created that contains the information about chemical modulators of epigenome reader domains recognizing chromatin epigenetic modifications [30]. Moreover, extensive studies of tumor associated DNA and histone modifications as well as of modifying enzymes led to the development and testing of multiple epigenetic medicines, especially directed against chromatin modifying or recognizing enzymes such as histone deacetylse inhibitors (HDACi) and DNA methyl transferases inhibitors (DNMTi) that are often used in combination [31-32].
Taken together, the epigenetic marks are of particular importance for cancer therapy as they could be used as diagnostic, prognostic and predictive markers in many types of the cancers including mainly lung and breast cancer, and epigenetic modulators, such as HDAC and DNMT, could be used as therapeutic targets for the effective cancer treatment.
Epigenetic regulation of the DNA repair gene expression is associated with the prognosis of chemotherapy resistance and aggressiveness of breast and lung cancer
It was shown that the level of DNA repair gene expression is associated with the chemotherapy resistance of different cancer types. For example, the expression level of DNA repair genes ERCC1 and BRCA1 is associated with chemotherapy resistance in non-small cell lung cancer cells (NSCLC) [33]. Recently, multiple data were obtained indicating that DNA and protein methylation as well as protein level of modifying enzymes correlate with chemotherapy resistance and aggressiveness of breast cancer (BC) [34] and lung cancer (LC) [35-36] and, therefore, they could be prognostic markers of these types of cancer. In particular, it was demonstrated that the overexpression of histone demethylase JARID1B/KDM5B led to the increase of the resistance to the cisplatine and doxorubicine in NSCLC [35]. It is worth to mention also that in several other types of cancers the changes in different histone demethylase expression levels influences the resistance and the aggressiveness of the oncological diseases. For example, the overexpression of the histone demethylase JARID1B/KDM5B in melanoma cells increases the resistance to chemotherapeutic compounds in vitro and in vivo[37-38]. It was shown also that the increase of JARID1B/KDM5B expression is associated with the chemotherapy resistance and worse prognosis of patients with epithelial ovarian cancer [39]. In several cases it is inferred thatthe decrease of the JARID1D/KDM5D histone demethylase quantity was correlated with the augmentation of docetaxel resistance at prostate cancer [40]. Over expression of another type of histone demethylases, Lysine-specific demethylase 1/KDM1, was associated with the increase of the chemotherapy resistance at liver cancer [41].
DNA repair and regulation in concordance with non-coding RNAs in lung cancer
LC is grossly classified as NSCLC or small cell lung cancer (SCLC) [42-44]. However, approximately 85% of lung cancers are NSCLCs with a reported 5-year survival rate is as low to 16%, a statistic which has not shown significant improvement over the last several decades [45]. A major reason behind this is the lack of early diagnosis as well as prompt prognosis of LC culminating in observable symptoms only at the advanced stage. Given the role that microRNAs (miRNAs) play in cell proliferation, cell cycle control, DNA damage repair, metabolism, apoptosis and drug resistance they become an attractive target of anti-cancer therapy including LC [46-47]. miRNAs influence the expression of their target genes acting as tumor suppressors and the oncogenes at LC [46]. In some instances it is related to DNA damage repair and can have an impact on chemo- and radio-resistance of this type of cancer [47-48]. Several miRNAs participate in BC and LC development and progression and are also used as their biomarkers (Figure 4). The patterns of the miRNAs partially overlap in these two types of cancer. MiRNAs that participate in breast and lung carcinogenesis are mostly involved in the multiple cellular processes whereas their functions and expression are differently regulated. For example, at LC the expression of miR-15a, miR-16 and miR-34a is decreased and this influence the cell cycle via cyclins D1, D2, E1 [49-50]. On the contrary, the expression miR-17 and miR-92a is increased due to the transcription regulation with c-MYC and this leads to the changes in cell proliferation and cancer development with the participation of PTEN, HIF-1a, CL2L11, CDKNA, TSP-1 [51-52]. Several other miRNAs including miR-29 and miR-548 being decreased in quantity influence cancer progression via epigenetic regulation of the gene expression involving DNA methyltransferases, DNMT-3A and DNMT-3B [53-54]. It is important to note that increased expression of miR-21, miR-221 and miR-222 confer the resistance of the lung tumor cells to inhibit tyrosine kinase [55-56].
Role of long non-coding RNAs (lncRNAs) in NSCLC has been discussed in detail in some studies [46-57]. Human transcription consists of a huge number of proteincoding mRNAs in addition to a large set of (ncRNAs) non-protein coding RNAs [58]. It has been discovered that the 87.3% of the human genome undergoes active transcription; however, less than 3% is translated in the form of proteins in actuality [59]. This discovery led to the revelation of wide expression of ncRNA. NcRNA is sub-divided into two categories: lncRNAs which are >200 bps in length and small ncRNAs which are <200 bps in length. LncRNAs are usually the non-protein coding transcripts and they are known to play a highly relevant role in the tumor development, metastasis and prognosis. A lot of studies have shown that the imbalance in regulation of lncRNAs is connected to development of LC. With the advent of new applications in bioinformatics and DNA sequencing analysis, it has been shown that lncRNAs interact with RNA, DNA and proteins and plays an important role in the initiation and development of the cancer. Many lncRNAs have been demonstrated to have a correlation with the drug resistance in LC in a specific p53–dependent manner. Furthermore, LncRNA-p21 (Trp53corl) is known to affect the expression of high number of gene targets that are usually expressed under the regulation of p53. In addition, lncRNA-p21 knockout mice have been shown to impose the G1/S checkpoint, which is known to increase cell division by inducing a decrease in Cdkn1aexpression [60]. LncRNA DDSR1, which is under the regulatory switch of ATM-NFkB pathway, has been shown to increase the ability of cellular DNA repair happened by the homologous recombination pathway via interaction with the BRCA1 and hnRNPUL1. Although, it needs to be verified if DDSR1 promotes cisplatin resistance only via DNA repair pathways [61]. P21, which is a cycle independent kinase inhibitor, is known to be induced by the p53 overexpression or after the DNA damage and thus it inhibits cell division or proliferation. EZH2 is also known to play a key role in modifications of DNA binding proteins, thereby it has a major role in regulation of global gene regulation via its interaction with lncRNA HOTAIR. Therefore, lncRNA HOTAIR is known to contribute to cisplatin resistance by decrease in p21 expression and its interaction with the EZH2, which results in chromosome modifications [62].
It has been shown [63] that there are significant differences in regulation of signaling pattern signature in NSCLC and SCLC. By studying the expression of 193 proteins in 34 in SCLC and 74 in NSCLC cell lines, PARP1 and EZH2 were found to be overexpressed in SCLC. It is also well reported that PARP inhibition downregulates RAD51 and BRCA1, which are key role players in the homologous recombination pathway. In addition, it was observed that the total and phosphorylated form of Rb (Retinoblastoma) were lower and expression level of E2F1 was higher in SCLC than in NSLC cell lines. In comparison, NSCLC cell lines exhibited a higher level of expression of tyrosine kinase receptors that show heterodimerization with EGFR, a group which includes AXL, MET and HER2 [64]. The gene expression levels of PARP1, DNA PKCs, BCL2, EZH2 and PCNA were comparatively higher in SCLC. The study documents a dichotomy in gene expression of NSCLC and SCLC. One of the key fascinating facts is that majority of the SCLC cell lines were observed to be sensitive to PARP inhibitors (e.g. AZD2281). However, NSCLC cell lines were observed to be resistant to PARP inhibition. It has also been shown that PARP inhibition leads to attenuation of expression of essential
It has been observed previously that PTEN loss and BRCA1/2 mutation serve as biomarkers for the PARP inhibition in the breast and ovarian cancer but their elucidation in LC development is not known. Almost 50% of human NSCLC cases are carriers of mitogenic cascade mutations i.e. RAS-RAF-MEK/ERK [63]. The signaling cascade is reported for activation of a target gene of the β-catenin, i.e. c-MYC [65]. Rapp et al. [66] have studied the ability of c-MYC in promotion of tumor formation and progress in a RAF-driven murine LC model. Also, MYC is an important candidate gene as it is reported to affect the reprogramming in many tumors, as per the earlier studies of interaction of RAF/MYC in murine hematopoietic system [67,68]. Hence, many research groups are keen on evaluating the role of the c-MYC in the mouse models of NSCLC. And it is found that dominant negative c-MYC mutant, endogenous c-MYC is known to play a significant role in non-metastatic K-Ras induced NSCLC [68]. It has also been shown that a transgenic model of c-MYC has synergistic effect with the mutant K-Ras in a tumor model to various degrees, however separate models did not show signs of metastasis [69,70]. Rapp et al. [66] have shown that either constitutive or inducible expression of the c-MYC along with that of c-RAF is needed for fast induction of metastasis in liver and lymph nodes. Thus, a combinatorial approach of co-expression of c-MYC and c-RAF transgenes results in a phenotypic switch to the alveolar columnar epithelial cells (APECs). These APECs belong to a class of fast growing tumor cell population that is also known to be dominant in induction of liver metastasis.
The role of chromatin remodeling and alternative splicing of DNA repair genes: their contribution to aggressiveness and tumor resistance
By combining exon and introns through splicing process of pre-mRNA a gene could lead to variety of transcripts that would explain the proteome and transcriptome diversity [71]. Alternative splicing can be managed by coupling transcription and chromatin modification and remodeling in response to the various signal transduction pathways that are activated by the external stimuli [16,17,20-72]. In contrast to common epigenetic control of the transcription initiation that takes place at gene promoter/enhancer regions, this epigenetic regulation of the alternative splicing mostly occurs at intragenic sites at the borders of exons/introns and is not necessarily accompanied with the same modification/remodeling events at the corresponding promoter regions [22-73]. Aberrant RNA splicing in cancer have been shown to be related to several genes which are implicated in the regulation of the hallmarks of cancer, as sustaining proliferation, evading cell death, angiogenesis and metastasis [74-75] and has an important application in the discovery of prognostic and predictive cancer biomarkes [1]. Studies have associated splicing variants to drug resistance and shortened patient survival in LC [76-78]. The tumor suppressor gene AIMP2 has shown to inhibit the proliferation of the cells and its regulation has been involved in tumor genesis. It was shown that splicing variant of this gene could compromise the activity of the AIMP2 playing a role in lung tumor induction [79]. In addition, it was demonstrated that AIMP2 regulates PARP-1 activation in neuronal model [80]. Interestingly, PARP-1 plays not only such a significant role in the DNA repair [81], but also it is important to modulate chromatin structure [82] and RNA polymerase movement during transcription and alternative splicing [83].
Variants of RNA splicing have also been related to aggressiveness and worse prognosis in BC [84-85]. Normal cell cycle regulator mdm2 is correlated to many tumors, it was demonstrated that an alternative splicing of this gene would shorten BC patient survival [86]. Mdm2 is well known by its capability to negatively regulate p53 implicating in proliferation and apoptotic inactivation, therefore, its over expression is present in many tumors [87]. Furthermore, the over expression of mdm2 was capable to inhibit the DNA double strand break repair [88], as well as capable to modify chromatin structure as reviewed by Wienken with co-authors [89]. Chromatin remodeling has been proved important to the pre-mRNA splicing. Several studies have investigated mutual link between chromatin and pre-mRNA processing after it has been shown by McCraken, [90] that RNA polymerase II enzyme couples transcription and prem RNA splicing. This link is mainly explained by two models, one is based on the physical interactions between the both i.e., RNA pol II and splicing factors [91-92]. The other is based on the transcription elongation rate [93-94]. As shown by Misteli and Spector [95], the C terminal portion of the RNA pol II reveled to be important for splicing factors to be recruited to sites of transcription. On the other hand, histones modifications can occur preferentially in exons, what supports a splicing marker mechanism [96]. Finally, splicing also demonstrated to be able to recruit methyl transferases and regulates histone methylation (Figure 5) [97-99].
Figure 5: Epigenetic regulation of alternative splicing. Mechanistically, changes in RNA polymerase II (RNA Pol II) elongation rate due to different histone modifications lead either to exon skipping upon the increase of RNA Pol II elongation rate, or to exon inclusion at the decrease of RNA Pol II elongation rate. The distinct profiles of alterations in the histones determine the maintenance or exclusion of an exon also via the recruitment of specific splicing factors, with or without the involvement of the binding of a chromatin-adaptor protein.
Interestingly, more than 7,690 different types of mutations of the BRCA1 gene have been reported worldwide, and it is spread over the entire length of the gene as per the Breast Cancer Information. Most of them lead to nonsense codons, missense variables or silent mutation and all the mutations can generate exon skipping. These variations act in the regulation of splicing by altering the recognition of splicing donor or acceptor sites in flanking regions. For example, (ESEs) exonicsplicing enhancers that are commonly present in the constitutive and alternative exons of some certain genes and are required for the efficient exon splicing are recognized by proteins rich in serine and arginine splicing factors (SRSF) [100-102]. Notably, SRSF3 (SRp20) is the smallest member of SRSF family involved with regulation and management of chromatin structure and function, plays important role in tumorigenesis and the altered expression of the SRSF3 is found in many human diseases and cancers. It is overexpressed in ovarian tumors and upregulated in cervical cancer, rhabdomyosarcoma and osteossarcoma, for example. Interestingly, SRSF3 knockdown generated expression-altered genes, upon homologous recombinationmediated (HRR) DNA repair, including BRCA1 and RAD51[103,104]. Still, in silico analyzes taken from (The Cancer Genome Atlas) TCGA project shows that SRSF3, XRCC2 ,BRCA1, RAD51and BLM are seen upregulated in ovarian tumors compared to normal tissues, showing the importance of HRR activations and interaction with splicing factors (eg. SRSF3) for development tumors and tumorigenicity maintenance[103].
It is described in the literature that SRSF3 associates with SRSF1 (responsible for genome integrity and maintenance) [105] and this binding marks the histone tail and chromatin that will be regulated by the phosphorylated serine 10 at histone 3 (H3S10P) thus generating chromosome condensation. In addition, it has been shown that H3S10P is dramatically reduced at increased DNA damage associated with inhibition of Aurora-B (chromosome passenger protein overexpressed in multiple tumor types) and PARP-1, showing that there is a correlation between damage in DNA, chromatin modification and regulation of mitotic events [106]. Presented studies demonstrate that in addition to the involvement in HRR in maintenance of genomic integrity to tumor suppression, doublestrand breaksare also important for activating the DNA repair of BRCAs (breast cancer susceptibility gene) family that include BRCA1 and BRCA2 [107]. Also, the cells those are deficient in BRCA proteins are 100 to 1000 fold more sensitive to the PARP (Poly(ADP-ribose)polymerase) inhibition compared to cells expressing BRCA [105]. The BRCA1-Δ11 splice variant (skipping of exon 11) is present in BC cell lines and stimulates the formation of tumors (in vivo assays) and inhibits the BRCA1 mediated doublestrand breaks repair. Thus, the induction of dual inhibition of BRCA1 and PARP activity is an attractive approach to treat BRCA1-associated breast tumors. In this context, it was observed that splice-switching oligonucleotides (SSOs) especially oligoAB perform the annealing to the premRNA sequences which manage splicing altering the RNA isoform formed at this treatment in BC cell lines and inhibiting the expression of the functional BRCA1 proteins. Also, in combination with PARP inhibiting, oligoAB induce increased cH2AX expression and death of breast tumor cell lines [108].
Conclusion
The data discussed in this article demonstrate the importance of the epigenetic regulation in cancer development and acquired resistance to the chemo- and the targeted therapy resistance. In these cases epigenetic regulation includes DNA and histone protein modifications, regulation with microRNAs and lncRNAs, and could occur at transcription initiation sites as well as during splicing events at intragenic regions comprising exon/intron boundaries. Emerging epigenetic marks could be used as prognostic and predictive markers of the aggressiveness and chemo- and targeted therapy resistance, respectively, of breast and lung cancers.
References
- Shelton BP, Misso NL, Shaw OM, Arthaningtyas E, Bhoola KD. (2008) Epigenetic regulation of human epithelial cell cancers. Curr Opin Mol Ther. 10(6):568-78.
- O'Leary K, Shia A, Schmid P. (2018) Epigenetic Regulation of EMT in Non-Small Cell Lung Cancer. Curr Cancer Drug Targets.18(1):89-96.
- Allis CD, Berger SL, Cote J, Dent S, Jenuwein T, et al. (2007) New nomenclature for chromatin-modifying enzymes. Cell. 131(4):633-36.
- Nakatani Y, Tagami H, Shestakova E. (2006) How is epigenetic information on chromatin inherited after DNA replication? Ernst Schering Res Found Workshop. 57:89-96.
- Shestakova E.A., Nakatani Y. (2015) Characterization of histone predeposition complexes from different cellular compartments. J. Investig Genomics. 2(1):1-4.
- Shestakova EA. (2015) Different mechanisms of epigenetic regulation of gene expression. MOJ Cell Sci. Rep. 2(1):1-5.
- Shestakova EA, Shematorova ЕК, Doludin YV, Shpakovski GV. (2019) Transcription factors and co-activators/co-repressors of transcription: structure-related cancer treatment. Nova Sci-ence Publishers. 72:175-223.
- Bogush TA, Shestakova EA, Vikhlyantseva NO, Bogush EA, ChemerisG Yu, et al. (2017) Epigenetic mechanisms of BRCA1 regulation. Oncogynecology. 23(2): 4–11.
- Shestakova EA. (2016) Epigenetic regulation of BRCA1 expression and its role in breast cancer stem cell development. Turk. J. Biol. 40(5):981-89.
- Scherbakov AM, Shestakova EA, Galeeva KE, Bogush ТА. (2019) BRCA1 and estrogen receptor α expression regulation in breast cancer cells. Mol. Biol. 53(3):442-51.
- Ohmoto A, Yachida S. (2017) Current status of poly(ADP-ribose) polymerase inhibitors and future directions. Onco Targets Ther. 10:5195-08.
- Du P, Zhang X, Liu H, Chen L. (2015) Lentivirus-Mediated RNAi silencing targeting ERCC1 reverses cisplatin resistance in cisplatin-resistant ovarian carcinoma cell line. DNA Cell Biol. 34(7):497-02.
- Scanlon SE, Glazer PM. (2015) Multifaceted control of DNA repair pathways by the hypoxic tumor microenvironment. DNA Repair (Amst). 32:180-89.
- Lu Y, Chu A, Turker MS, Glazer PM. (2011) Hypoxia-induced epigenetic regulation and silencing of the BRCA1 promoter. Mol. Cell. Biol. 31(16):3339–50.
- Wu ZQ, Li XY, Hu CY, Ford M, Kleer CG, et al. (2012) Canonical Wnt signaling regulates Slug activity and links epithelial-mesenchymal transition with epigenetic Breast Cancer 1, Early Onset (BRCA1) repression. Proc. Natl. Acad. Sci. USA. 109(41):16654–659.
- Luco RF, Allo M, Schor IE, Kornblihtt AR, Misteli T. (2011) Epigenetics in alternative pre-mRNA splicing. Cell. 144(1):16-26.
- Zhu LY, Zhu YR, Dai DJ, Wang X, Jin HC. (2018) Epigenetic regulation of alternative splicing. Am J Cancer Res. 8(12):2346-58.
- Davie JR, Xu W, Delcuve GP. (2016) Histone H3K4 trimethylation: dynamic interplay with pre-mRNA splicing. Biochem Cell Biol. 94(1):1-11.
- Saint-Andre V, Batsche E, Rachez C, Muchardt C. (2011) Histone H3 lysine 9 trimethylation and HP1γ favor inclusion of alternative exons. Nat Struct Mol Biol. 18(3):337-44.
- Luco RF, Pan Q, Tominaga K, Blencowe BJ, Pereira-Smith OM, et al. (2010) Regulation of alternative splicing by histone modifications. Science. 327(5968):996-00.
- Allo M, Buggiano V, Fededa JP, Petrillo E, Schor I, et al. (2009) Control of alternative splicing through siRNA-mediated transcriptional gene silencing. Nat Struct Mol Biol. 16(7):717-24.
- Schor IE, Kornblihtt AR. (2009) Playing inside the genes: Intragenic histone acetylation after membrane depolarization of neural cells opens a path for alternative splicing regulation.Commun Integr Biol. 2(43):341-3.
- Batsche E, Yaniv M, Muchardt C. (2006) The human SWI/SNF subunit Brm is a regulator of alternative splicing. Nat Struct Mol Biol. 13(1):22-29.
- Ameyar-Zazoua M, Rachez C, Souidi M, Robin P, Fritsch L, et al. (2012) Argonaute proteins couple chromatin silencing to alternative splicing. Nat Struct Mol Biol. 19(10):998-04.
- Cedar H, Bergman Y. (2009) Linking DNA methylation and histone modification: patterns and paradigms. Nat Rev Genet. 10(5):295-04.
- Rose NR, Klose RJ. (2014) Understanding the relationship between DNA methylation and histone lysine methylation. Biochim. Biophys. Acta., 1839(12):1362-72.
- Thinnes CC, England KS, Kawamura A, Chowdhury R, Schofield CJ, et al. (2014) Targeting histone lysine demethylases - progress, challenges, and the future. Biochim Biophys Acta. 1839(12):1416-32.
- Sanchez R, Meslamani J, Zhou MM. (2014) The bromodomain: from epigenome reader to druggable target. 1839(8):676-85.
- Mertz JA, Conery AR, Bryant BM, Sandy P, Balasubramanian, S, et al. (2011) Targeting MYC dependence in cancer by inhibiting BET bromodomains. Proc. Natl Acad Sci USA. 108(40):16669-674.
- Meslamani J, Smith SG, Sanchez R, Zhou MM. (2014) ChEpiMod: a knowledgebase for chemical modulators of epigenome reader domains. Bioinformatics. 30(10):1481-83.
- Eckschlager T, Plch J, Stiborova M, Hrabeta J. (2017) Histone deacetylase inhibitors as anticancer drugs. Int. J. Mol. Sci. 18(7):1414 (p. 1-25).
- Stiborova M, Eckschlager T, Poljakova J, Hrabeta J, Adam V, et al. (2012) The synergistic effects of DNA-targeted chemotherapeutics and histone deacetylase inhibitors as therapeutics strategies for cancer treatment. Curr. Med. Chem. 19(25):4218-38.
- Wang S, Liu F, Zhu J, Chen P, Liu H, et al. (2016) DNA repair genes ERCC1 and BRCA1 expression in non-small cell lung cancer chemotherapy drug resistance. Med Sci Monit. 22:1999-05.
- Damiano V, Brisotto G, Borgna S, di Gennaro A, Armellin M, et al. (2017) Epigenetic silencing of miR-200c in breast cancer is associated with aggressiveness and is modulated by ZEB1. Genes Chromosomes Cancer. 56(2):147-58.
- Kuo KT, Huang WC, Bamodu OA, Lee WH, Wang CH, et al. (2018) Histone demethylase JARID1B/KDM5B promotes aggressiveness of non-small cell lung cancer and serves as a good prognostic predictor. Clin Epigenetics. 10(1):107.
- Ávila-Moreno F, Armas-López L, Álvarez-Moran AM, López-Bujanda Z, Ortiz-Quintero B, et al. (2014) Over expression of MEOX2 and TWIST1 is associated with H3K27me3 levels and determines lung cancer chemoresistance and prognosis. PLoS One. 9(12):e114104.
- Roesch A, Vultur A, Bogeski I, Wang H, Zimmermann KM, et al. (2013) Overcoming intrinsic multidrug resistance in melanoma by blocking the mitochondrial respiratory chain of slow-cycling JARID1B(high) cells. Cancer Cell, 23(6):811-25.
- Zubrilov I, Sagi-Assif O, Izraely S, Meshel T, Ben-Menahem S, et al. (2015) Vemurafenib resistance selects for highly malignant brain and lung-metastasizing melanoma cells. Cancer Lett. 361(1):86-96.
- Wang L, Mao Y, Du G, He C, Han S. (2015) Overexpression of JARID1B is associated with poor prognosis and chemotherapy resistance in epithelial ovarian cancer. Tumour Biol. 36(4):2465-72.
- Komura K, Jeong SH, Hinohara K, Qu F, Wang X, et al. (2016) Resistance to docetaxel in prostate cancer is associated with androgen receptor activation and loss of KDM5D expression. Proc Natl Acad Sci USA. 113(22):6259-64.
- Lei ZJ, Wang J, Xiao HL, Guo Y, Wang T, et al. (2015) Lysine-specific demethylase 1 promotes the stemness and chemoresistance of Lgr5(+) liver cancer initiating cells by suppressing negative regulators of β-catenin signaling. 34(24):3188-98.
- Singhavi H, Ahluwalia JS, Stepanov I, Gupta PC, Gota V, et al. (2018) Tobacco carcinogen research to aid understanding of cancer risk and influence policy. Laryngoscope Investig Otolaryngol. 3(5):372-376.
- Zhang ZY, Fu SL, Xu SQ et al. By downregulating Ku80, hsa-miR-526b suppresses non-small cell lung cancer. Oncotarget 2015; 6: 1462–77.
- Zhang HH, Pang M, Dong W, Xin JX, Li YJ, et al. (2014) miR-511 induces the apoptosis of radioresistant lung adenocarcinoma cells by triggering BAX. Oncol Rep. 31(3):1473–9.
- Gao F, Chang J, Wang H, Zhang G. (2014) Potential diagnostic value of miR-155 in serum from lung adenocarcinoma patients. Oncol Rep. 31(1): 351–7.
- Fortunato O, Boeri M, Verri C, Moro M, Sozzi G. (2014) Therapeutic use of microRNAs in lung cancer. Biomed Res Int. 2014:756975.
- Guo QR, Wang H, Yan YD, Liu Y, Su CY, et al. (2020) The Role of Exosomal microRNA in Cancer Drug Resistance. Front Oncol. 10:472.
- Li L, Zhu T, Gao YF, Zheng W, Wang CJ, et al. (2016) Targeting DNA Damage Response in the Radio(Chemo)therapy of Non-Small Cell Lung Cancer. Int J Mol Sci. 17(6):839.
- Bandi N, Zbinden S, Gugger M, Arnold M, Kocher V, et al. (2009) miR-15a and miR-16 are implicated in cell cycle regulation in a Rb-dependent manner and are frequently deleted or down-regulated in non-small cell lung cancer. Cancer Res. 69(13):5553-9.
- Bandi N, Vassella E. (2011) miR-34a and miR-15a/16 are co-regulated in non-small cell lung cancer and control cell cycle progression in a synergistic and Rb-dependent manner. Mol Cancer. 10:55.
- Matsubara H, Takeuchi T, Nishikawa E, Yanagisawa K, Hayashita Y, et al. (2007) Apoptosis induction by antisense oligonucleotides against miR-17-5p and miR-20a in lung cancers over expressing miR-17-92. Oncogene. 26(41):6099-105.
- O'Donnell KA, Wentzel EA, Zeller KI, Dang CV, Mendell JT. (2005) c-Myc-regulated microRNAs modulate E2F1 expression. Nature. 435(7043):839-43.
- Fabbri M, Garzon R, Cimmino A, Liu Z, Zanesi N, et al. (2007) MicroRNA-29 family reverts aberrant methylation in lung cancer by targeting DNA methyltransferases 3A and 3B. Proc Natl Acad Sci USA. 104(40):15805-10.
- Hu B, Ying X, Wang J, Piriyapongsa J, Jordan IK, et al. (2014) Identification of a tumor-suppressive human-specific microRNA within the FHIT tumor-suppressor gene. Cancer Res. 74(8):2283-94.
- Garofalo M, Romano G, Di Leva G, Nuovo G, Jeon YJ, et al. (2011) EGFR and MET receptor tyrosine kinase-altered microRNA expression induces tumorigenesis and gefitinib resistance in lung cancers. Nat Med. 18(1):74-82.
- Li B, Ren S, Li X, Wang Y, Garfield D, et al. (2014) MiR-21 overexpression is associated with acquired resistance of EGFR-TKI in non-small cell lung cancer. Lung Cancer. 83(2):146-53.
- Wang KC, Chang HY. (2011) Molecular Mechanisms of Long Noncoding RNAs. Mol Cell. 43(6):904-14.
- Siegel RL, Miller KD, Jemal A. (2016) Cancer statistics, 2016. CA Cancer J Clin. 66:7–30.
- Rom UA, Derrien T, Beringer M, Gummireddy K, Gardini A, et al. (2010) Long noncoding RNAs with enhancer-like function in human cells. Cell 143(1):46–58.
- Djebali S, Davis CA, Merkel A, Dobin A, Lassmann T, et al. (2012) Landscape of transcription in human cells. Nature. 489(7414):101–8.
- Dimitrova N, Zamudio JR, Jong RM, Soukup D, Resnick R, et al. (2014) LincRNA-p21 activates p21 in cis to promote Polycomb target gene expression and to enforce the G1/S checkpoint. Mol Cell. 54(5):777–90.
- Sharma V, Khurana S, Kubben N, Abdelmohsen K, Oberdoerffer P, et al. (2015) A BRCA1-interacting lncRNA regulates homologous recombination. EMBO Rep. 16(11): 1520–34.
- Byers LA, Wang J, Nilsson MB, Fujimoto J, Saintigny P, et al. (2012) Proteomic profiling identifies dysregulated pathways in small cell lung cancer and novel therapeutic targets including PARP1 . Cancer Discov. 2(9):798–11.
- Liu Z, Sun M, Lu K, Liu J, Zhang M, et al. (2013) The long noncoding RNA HOTAIR contributes to cisplatin resistance of human lung adenocarcinoma cells via downregualtion of p21 (WAF1/CIP1) expression. PLoS ONE. 8(10): e77293.
- Marks JL, McLellan MD, Zakowski MF, Lash AE, Kasai Y, et al. (2007) Mutational analysis of EGFR and related signaling pathway genes in lung Adenocarcinomas identifies a novel somatic kinase domain mutation in FGFR4. PLoS ONE. 2:e426.
- Rapp UR, Korn C, Ceteci F, Karreman C, Luetkenhaus K, et al. (2009) Myc is a metastasis gene for non-small-cell lung cancer. PLoS One. 4(6): e6029.
- Kerkhoff E, Rapp UR. (1998) Cell cycle targets of Ras/Raf signalling. Oncogene 17(11 Reviews):1457–62.
- Rapp UR, Ceteci F, Schreck R. (2008) Oncogene-induced plasticity and cancer stem cells. Cell Cycle. 7(1):45–51.
- Soucek L, Lawlor ER, Soto D, Shchors K, Swigart LB, et al. (2007) Mast cells are required for angiogenesis and macroscopic expansion of Myc-induced pancreatic islet tumors. Nat Med 13(10): 1211–18.
- Tran PT, Fan AC, Bendapudi PK, Koh S, Komatsubara K, et al. (2008) Combined Inactivation of MYC and K-Ras oncogenes reverses tumorigenesis in lung adenocarcinomas and lymphomas. PLoS ONE 3(5): e2125.
- Nilsen TW, Graveley BR. (2010) Expansion of the eukaryotic proteome by alternative splicing. Nature. 463(7280):457–63.
- Luco RF, Misteli T. (2011) More than a splicing code: integrating the role of RNA, chromatin and non-coding RNA in alternative splicing regulation. Curr Opin Genet Dev. 21(4):366-72.
- Schor IE, Rascovan N, Pelisch F, Allo M, Kornblihtt AR. (2009) Neuronal cell depolarization induces intragenic chromatin modifications affecting NCAM alternative splicing. Proc Natl Acad Sci USA. 106(11):4325-30.
- Ladomery M. (2013) Aberrant Alternative Splicing Is Another Hallmark of Cancer. Int J Cell Biol. 2013:463786.
- Sveen A, Kilpinen S, Ruusulehto A, Lothe RA, Skotheim RI. (2016) Aberrant RNA splicing in cancer; Expression changes and driver mutations of splicing factor genes. Oncogene. 35(19):2413–27.
- Bria E, Di Modugno F, Sperduti I, Iapicca P, Visca P, et al. (2014) Prognostic impact of alternative splicing-derived hmena isoforms in resected, node-negative, non-small-cell lung cancer. Oncotarget, 5(22):11054–063.
- Planque C, Choi YH, Guyetant S, Heuzé-Vourc'h N, Briollais L, et al. (2010) Alternative splicing variant of kallikrein-related peptidase 8 as an independent predictor of unfavorable prognosis in lung cancer. Clin Chem. 56(6):987–97.
- Ng KP, Hillmer AM, Chuah CT, Juan WC, Ko TK, et al. (2012) A common BIM deletion polymorphism mediates intrinsic resistance and inferior responses to tyrosine kinase inhibitors in cancer. Nat Med. 18(4):521–28.
- Choi JW, Kim DG, Lee AE, Kim HR, Lee JY, et al. (2011) Cancer-associated splicing variant of tumor suppressor AIMP2/p38: pathological implication in tumorigenesis. PLoS Genet. 7(3):e1001351.
- Lee Y, Karuppagounder SS, Shin JH, Lee YI, Ko HS, et al. (2013) Parthanatos Mediates AIMP2 Activated Age Dependent Dopaminergic Neuronal Loss. Nat Neurosci., 16(10);1392-1400.
- Ray Chaudhuri A, Nussenzweig A. (2017) The multifaceted roles of PARP1 in DNA repair and chromatin remodelling. Nat Rev Mol Cell Biol. 18(10):610–21.
- Kim MY, Mauro S, Gévry N, Lis JT, Kraus WL. (2004) NAD+-dependent modulation of chromatin structure and transcription by nucleosome binding properties of PARP-1. Cell. 119(6):803–14.
- Matveeva EA, Al-Tinawi QMH, Rouchka EC, Fondufe-Mittendorf YN. (2019) Coupling of PARP1-mediated chromatin structural changes to transcriptional RNA polymerase II elongation and cotranscriptional splicing. Epigenetics Chromatin. 12(1):15.
- Végran F, Boidot R, Oudin C, Riedinger JM, Bonnetain F, et al. (2006) Overexpression of caspase-3s splice variant in locally advanced breast carcinoma is associated with poor response to neoadjuvant chemotherapy. Clin Cancer Res. 12(19):5794–800.
- Zhao S, Chang SL, Linderman JJ, Feng FY, Luker GD. (2014) A Comprehensive Analysis of CXCL12 Isoforms in Breast Cancer. Transl Oncol. 7(3):429–38.
- Lukas J, Gao DQ, Keshmeshian M, Wen WH, Tsao-Wei D, et al. (2001) Alternative and aberrant messenger rna splicing of the mdm2 oncogene in invasive breast cancer. Cancer Res. 61(7):3212–19.
- Iwakuma T, Lozano G. (2003) MDM2 , An Introduction. 1(14):993–000.
- Alt JR, Bouska A, Fernandez MR, Cerny RL, Xiao H, et al. (2005) Mdm2 binds to Nbs1 at sites of DNA damage and regulates double strand break repair. J Biol Chem. 280(19): 18771–781.
- Wienken M, Moll UM, Dobbelstein M. (2017) Mdm2 as a chromatin modifier. J Mol Cell Biol. 9(1):74–80.
- McCracken S, Fong N, Yankulov K, Ballantyne S, Pan G, et al. (1997) The C-terminal domain of RNA polymerase II couples mrna processing to transcription. Nature. 385(23): 357–61.
- De la Mata M, Kornblihtt AR. (2006) RNA polymerase II C-terminal domain mediates regulation of alternative splicing by srp20. Nat Struct Mol Biol. 13(11):973–80.
- Furger A, O'Sullivan JM, Binnie A, Lee BA, Proudfoot NJ. (2002) Promoter proximal splice sites enhance transcription. Genes Dev. 16(21):2792–99.
- De la Mata M, Alonso CR, Kadener S, Fededa JP, Blaustein M, et al. (2003) A slow RNA polymerase II affects alternative splicing in vivo. Mol Cell. 12(2):525–32.
- Nogues G, Kadener S, Cramer P, Bentley D, Kornblihtt AR. (2002) Transcriptional activators differ in their abilities to control alternative splicing. J Biol Chem. 277(45):43110–114.
- Misteli T, Spector DL. (1999) RNA polymerase II targets pre-mRNA splicing factors to transcription sites in vivo. Mol cell. 3(6):697–05.
- Kolasinska-Zwierz P, Down T, Latorre I, Liu T, Liu XS, et al. (2009) Differential chromatin marking of introns and expressed exons by h3k36me3. Nat Genet. 41(3):376–81.
- De Almeida SF, Grosso AR, Koch F, Fenouil R, Carvalho S, et al. (2011) Splicing enhances recruitment of methyltransferase HYPB/Setd2 and methylation of histone H3 Lys36. Nat Struct Mol Biol. 18(9):977–83.
- Kim S, Kim H, Fong N, Erickson B, Bentley DL. (2011) Pre-mrna splicing is a determinant of histone H3K36 methylation. Proc Natl Acad Sci U S A. 108(33):13564–569.
- Zhou HL, Hinman MN, Barron VA, Geng C, Zhou G, et al. (2011) Hu proteins regulate alternative splicing by inducing localized histone hyperacetylation in an RNA-dependent manner. Proc Natl Acad Sci U S A. 108(36):627–35.
- Brandão RD, van Roosendaal K, Tserpelis D, Gómes Garcia E, Blok MJ. (2011) Characterisation of unclassified variants in the BRCA1/2 genes with a putative effect on splicing. Breast Cancer Res Treat. 3(129):971:82.
- Liu HX, Cartegni L, Zhangi MQ, Krainer AR. (2001) A mechanism for exon skipping caused by nonsense or missense mutations in BRCA1 and other genes. Nat Gene. 27(1):55-58.
- Mazoyer S, Puget N, Perrin-Vidoz Laure, Lynch HT, Serova-Sinilnikova OM, et al. (1998) A BRCA1 Nonsense Mutation Causes Exon Skipping. Am J Hum Genet. 62(3):713-15.
- He X, Zhang P. (2015) Serine/arginine-rich splicing factor 3 (SRSF3) regulates homologous recombination-mediated DNA repair. Mol Cancer. 14:158.
- Ajiro M, Jia R, Yang Y, Zhu J, Zheng, ZM. (2016) A genome landscape of SRSF3- regulated splicing events and gene expression in human osteosarcoma U2OS cells. Nucleic Acids Res. 44(4):1854:70.
- Loomis RJ, Naoe Y, Parker JB, Savic V, Bozovsky R, et al. (2010) Chromatin Binding of SRp20 and ASF/SF2 and Dissociation from Mitotic Chromosomes Is Modulated by Histone H3 Serine 10 Phosphorylation. Mol Cell. 33(4):450-61.
- Monaco L, Kolthur-Seetharam U, Loury R, Ménissier-De Murcia J, De Murcia G, et al. (2005) Inhibition of Aurora-B kinase activity by poly(ADP-ribosyl)ation in response to DNA damage. Proc Natl Acad Sci U S A. 102(40):14244:248.
- Yoshida K, Miki Y. (2004) Role of BRCA1 and BRCA2 as regulators of DNA repair, transcription, and cell cycle in response to DNA damage. Cancer Sci. 95(11) :866-71.