The Effects of Stem Cells on Acute Ischemic Strokes
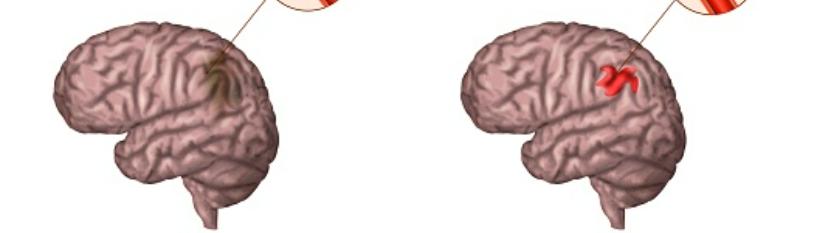
Abigail Stock1 and Vincent S. Gallicchio2*
1Department of Biological Sciences, College of Science, Clemson University, Clemson, South, Carolina, USA 20636 2Department of Biological Sciences College of Science 122 Long Hall Clemson University Clemson, SC 29636
*Corresponding author: Vincent S Gallicchio, Department of Biological Sciences College of Science 122 Long Hall Clemson University Clemson, SC 29636.
Citation: Stock A, Gallicchio VS. (2023) The Effects of Stem Cells on Acute Ischemic Strokes. J Stem Cell Res. 4(1):1-15.
Received: April 28, 2023 | Published: May 13, 2023
Copyright© 2023 genesis pub by Stock A, et al. CC BY NC-ND 4.0 DEED. This is an open-access article distributed under the terms of the Creative Commons Attribution-NonCommercial-No Derivatives 4.0 International License., This allows others distribute, remix, tweak, and build upon the work, even commercially, as long as they credit the authors for the original creation.
DOI: https://doi.org/10.52793/JSCR.2023.4(1)-46
Abstract
A stroke is a neurological disease that causes damage to the brain due to an obstruction of blood supply. Stroke prevalence has shown a drastic increase as the world’s population ages. The effects of a stroke can lead to long-lasting brain damage, long-term disability, or death. Current treatment options are limited, and their efficacy is dependent on the element of time. However, stem cell therapy may provide an alternative means of treatment for stroke patients. The ability of mesenchymal stem cells to self-renew and differentiate into multiple different cell types, including neurons and glial cells, shows promise for stroke treatment. Therapeutically, MSCs are capable of diminishing inflammation through immunomodulation, promoting therapeutic effects through the release of trophic factors, inducing angiogenesis, increasing the proliferation of neuroblasts, and promoting the replacement of damaged cells. Preclinically and clinically, MSC therapy appears to be safe; however, treatment efficacy has yet to be established.
Keywords
Stroke; Stem cells; Ischemic stroke; Clinical trials; Mesenchymal stem cells.
List of Abbreviations
IS: Ischemic stroke
MSC: Mesenchymal stem cell
TOAST: Trial of Acute Stroke Treatment
mRS: modified Rankin scale
NIHSS: National Institutes of Stroke Scale
BI: Barthel Index
tPA: tissue plasminogen activator
IV: intravenous
IC: intracerebral
ASC: Adipose-derived stem cell
Introduction
A stroke is a neurological disease that occurs in response to a blockage in blood flow to the brain [1]. As a result, the brain is unable to receive adequate amounts of oxygen and nutrients, which leads to cell death within minutes. A stroke can also be caused by sudden bleeding in the brain if there is damage to the cells. The effects of a stroke can produce lasting brain damage, long-term disability, or death. Mild symptoms associated with a stroke include a sudden and severe headache, trouble seeing, sudden weakness, or trouble understanding speech or speaking [1].
Stroke is a leading cause of death and disability globally. Over the last decade, the incidence of stroke has increased to 85-95 per 100,000 individuals. However, the incidence increases to 1151-1216 per 100,000 for individuals over the age of 75 [2]. As the number of individuals aged 65+ continues to increase, the incidence of strokes is expected to mimic this rise [3]. Despite differing contributions to different subtypes of stroke, hypertension remains the most significant modifiable risk factor [2]. As individuals increase in age, the risk of having a stroke rapidly increases. After the age of 55, each decade the incidence of stroke doubles [4]. Neuroimaging has become the primary detection method in modern clinical practice to determine the precise pattern of tissue damage [2]. There are restrictions for the type of neuroimaging used depending on the accessibility of certain methods, contraindications, or a patient’s ability to receive thrombolysis [5]. Non contrast computed tomography (CT) and magnetic resonance imaging (MRI) are typical neuroimaging techniques used for all patients with stroke symptoms. These neuro-imaging processes are used to differentiate between ischemic and hemorrhagic stroke, while also excluding the possibility of non ischemic central nervous system lesions. Acute ischemic stroke is best detected by an MRI due to increased resolution capabilities and sensitivity. However, non-contrast CT is a quicker and more affordable option [5].
Figure 1: The Algorithm for diagnosis of acute stroke [5].
Stroke is distinguished by two main forms: ischemic and hemorrhagic. Most strokes (87%) are ischemic, which are caused by artery blockages. The other 13% of strokes occur due to an intracerebral hemorrhage [3]. Various pathophysiologic mechanisms are responsible for the manifestation of ischemic and hemorrhagic strokes in neurological deficits. The cause of an ischemic stroke is typically the occlusion of a single blood vessel. The blockade induces a deprivation of glucose and oxygen in the brain, which the collateral vessels in the brain are unable to compensate for [1,6]. There are two distinct damaged areas in the brain that are affected by inadequate blood supply, specifically the ischemic core and the penumbra. The ischemic core is susceptible to irreversible damage and death caused by necrosis because it does not meet the threshold of blood flow required for cell survival. However, brain cells may recover in the penumbra if the blood flow is returned to normal in time. The mechanism that prevents cells from immediate death is the minimal energy provided by the blood flow in the penumbra. Nevertheless, neurological functions are not supported by the minimal flow of blood [7]. Because of the ability of the penumbra to escape cell death in adequate time, current treatments involving stem cell transplantation focus on preventing the penumbra from death or aim to establish a new neuronal network [8].
In the early 1990s, the Trial of Acute Stroke Treatment (TOAST) classification system was established to categorize ischemic strokes into five subtypes. The underlying pathophysiological mechanisms included large-artery atherosclerosis, cardioembolism, small vessel occlusion, a stroke of other determined etiology, and a stroke of undetermined etiology [9]. Large-artery atherosclerosis can be described as thrombotic or embolic. A thrombotic stroke occurs when a blood clot forms locally in the brain, whereas an embolic stroke is caused by a clot in another location in the body that travels to the brain vasculature [11]. An automated version of classification has since been developed, the SSS-TOAST, the Causative Classification System (CCS), as an evidence-based classification system. The system utilizes a questionnaire-style classification scheme for clinical and diagnostic evaluations of ischemic stroke [10].
Large-artery atherosclerosis (embolus/thrombosis) |
Cardioembolism (high-risk/medium-risk) |
Small-vessel occlusion (lacune) |
Stroke of other determined etiology |
Stroke of undetermined etiology |
a. Two or more causes identified |
b. Negative evaluation |
c. Incomplete evaluation |
Treatment
Acute ischemic stroke detection is predicated on the element of time. The typical patient experiences a loss of 1.9 million neurons for each minute the stroke remains untreated [12]. Numerous functional classification systems have been established to guide logical acute management, examination, and secondary prevention [2]. The modified Rankin scale (mRS) is a commonly used classification system for stroke patients. The 7-point scale is a measure of global disability and is used to evaluate the potential outcomes of stroke patients [13]. However, it has practical limitations because it requires an additional assessment, around 3 months, once patients are given the opportunity to resume daily activities. The time span increases the risk that individuals may not follow-up [14].
Grade |
mRS |
0 |
No symptoms |
1 |
No significant disability: despite symptoms, able to carry out all usual duties and activities |
2 |
Slight disability: unable to perform all previous activities but able to look after own affairs without assistance |
3 |
Moderate disability: requiring some help but able to walk without assistance |
4 |
Moderately severe disability: unable to walk without assistance and unable to attend to own bodily needs without assistance |
5 |
Severe disability: bedridden, incontinent, and requiring constant nursing care and attention |
6 |
Death |
Another evaluation method is the National Institutes of Health Stroke Scale (NIHSS). The treatment strategies utilized for strokes are based on detection tools derived from the NIHSS, which focuses on acute stroke recognition. The 42-point systematic assessment tool is employed by emergency medical services in the prehospital setting and should be conducted within 10 minutes of arrival [15]. The treatment strategy for acute stroke is based on the evaluation and classification of the stroke severity and is used to predict prognosis [5]. Unlike the mRS, the NIHSS does not measure functional outcomes but neurological deficits. More severe neurological deficit is observed in scores closer to 42 [14].
Category |
Scale Definition |
Score |
|
1A |
Level of Consciousness |
Alert |
0 |
Drowsy |
1 |
||
Stuporous |
2 |
||
Coma |
3 |
||
1B |
LOC – Questions |
Answers both questions correctly |
0 |
Answers one question correctly |
1 |
||
Answers neither question correctly |
2 |
||
1C |
LOC – Commands |
Performs both tasks correctly |
0 |
Performs one task correctly |
1 |
||
Performs neither task correctly |
2 |
||
2 |
Best Gaze |
Normal |
0 |
Partial gaze palsy |
1 |
||
Forced deviation |
2 |
||
3 |
Visual |
No visual loss |
0 |
Partial hemianopia |
1 |
||
Complete hemianopia |
2 |
||
Bilateral hemianopia (blind including cortical blindness) |
3 |
||
4 |
Facial Palsy |
Normal symmetrical movements |
0 |
Minor paralysis |
1 |
||
Partial paralysis |
2 |
||
Complete paralysis |
3 |
||
5 |
Motor Arm |
No drift |
0 |
Drift |
1 |
||
Some effort against gravity |
2 |
||
No movement |
3 |
||
6 |
Motor Leg |
No drift |
0 |
Drift |
1 |
||
Some effort against gravity |
2 |
||
No movement |
3 |
||
7 |
Limb Ataxia |
Absent |
0 |
Present in one limb |
1 |
||
Present in two limbs |
2 |
||
8 |
Sensory |
Normal; no sensory loss |
0 |
Mild-to-moderate sensory loss |
1 |
||
Severe to total sensory loss |
2 |
||
9 |
Best Language |
No aphasia; normal |
0 |
Mild-to-moderate aphasia |
1 |
||
Severe aphasia |
2 |
||
Mute, global aphasia |
3 |
||
10 |
Dysarthria |
Normal |
0 |
Mild-to-moderate dysarthria |
1 |
||
Severe dysarthria |
2 |
||
11 |
Extinction and Inattention |
No abnormality |
0 |
Visual, tactile, auditory, spatial, or personal inattention |
1 |
||
Profound hemi-inattention or extinction to more than one modality |
2 |
||
Total Score = 0 to 42 |
Table 3: The National Institutes of Health Stroke Scale (NIHSS).
Current ischemic stroke therapeutic interventional treatments are limited. Treatment options are primarily restricted to tissue plasminogen activator (tPA) and mechanical thrombectomy [16]. When activated platelets cluster together onto fibrin meshes, the formation of blood clots occurs. The dissolution of fibrin meshes is catalyzed by a broad-spectrum protease, plasmin. It appears in the blood as a plasminogen, which is an inactive zymogen. The tissue plasminogen activator (tPA) works by generating an adequate amount of plasmin from plasminogen in order to successfully break down the fibrin [11]. tPA is used sparingly on account of complications that the drug may cause, including the transformation of ischemic to hemorrhage. Furthermore, tPA has poor revascularization rates and a short efficacy window [17]. However, tPA is being considered as a key therapeutic target rather than just a therapeutic means in stroke therapy [11]. Another method of treatment is a mechanical thrombectomy, which utilizes an endovascular catheter and other devices to remove or break up the thrombus that is obstructing an intracranial artery [18]. Mechanical thrombectomy is currently the standard treatment utilized for acute ischemic stroke patients that suffer from a large vessel occlusion [17].
Stem Cell Therapy
Studies have shown that stem cell therapy may provide functional improvement for ischemic stroke. During the acute phase of stroke, stem cells are intended to preserve neural tissue. Replenishment of lost tissue is sought after in the chronic stage [19]. In animal stroke models, various types of stem cells have improved neurological outcomes. The success in animal models has encouraged researchers to pursue stem cells as potential therapeutic targets [7]. Stem cells can differentiate into specific cell types, which supports their potential use for tissue regeneration and repair. Stem cells are also capable of self-renewal. These cells may be classified into two major classes: pluripotent and multipotent. Pluripotent stem cells can differentiate into any cell in the adult body. They are present in the embryo; however, their presence is limited before they transform into more specialized cells, known as multipotent stem cells. Multipotent stem cells organize themselves into several subtypes that may arise from a particular germline (endoderm, mesoderm, ectoderm) or tissue [20].
Stem cell intervention has been established as a safe method of treatment for stroke patients. Preclinical research has proven that there is ample evidence to support the conduction of clinical trials. However, efficacy is still considered uncertain [21]. Stem cell therapy aims to augment neuro regeneration and decrease the deficits inflicted by stroke [22].
Mesenchymal Stem Cells
Mesenchymal stem cells (MSCs) can self-renew and differentiate into multiple different cell types under specific conditions, including neurons, glial cells, adipocytes, chondrocytes, and osteoblasts [23]. There are multiple different delivery methods for MSCs. In both animal and clinical research, intravenous injection (IV) and intracerebral injection (IC) are the most common. IV delivery is the most effective option for large infarctions; however, few cells can reach the site of injury. IC is more effective at delivering the drugs directly to the target area, but there is an increased risk of intracranial hypertension, nerve damage, and functional lesions [23]. The therapeutic mechanisms of MSCs involve the attenuation of inflammation through immunomodulation, the release of trophic factors to promote therapeutic effects, the induction of angiogenesis, the proliferation of neuroblasts, and the replacement of damaged cells [24]. Preclinically and clinically, MSC therapy appears to be safe; however, the efficacy of treatment has yet to be established [16].
Mesenchymal stem cells are chosen due to their potential in enhancing functional recovery and improving injured brain function caused by a stroke. The ability of MSCs to promote recovery is contributed to their paracrine effect, which is seen through immune regulation. Due to the interactions between MSCs and immune cells, they can control and enhance destructive inflammatory responses. Furthermore, angiogenesis is promoted through secreted MSC nutritional factors, which include growth factors, enzymes, matrix metalloproteinases, and adhesion molecules [19]. Adipose-derived stem cells (ASCs) show potential in damaged tissue and organ regeneration. ASCs are a type of MSC and can therefore differentiate into various cell lineages [25]. In comparison to bone marrow-derived MSCs, ASCs can produce a higher number of cells because there is an abundance of adipose tissue [26]. ASCs express cytokines, which establishes them as a potential therapeutic target for transplantation and ischemia patients. Furthermore, ASC tissue regeneration is more effective because they are responsive to growth factors as well [26]. Through the production of growth factors, ASCs also stimulate angiogenesis. In vivo, ASCs have shown promise in the improvement of vessel formation and blood perfusion, which establishes ASCs as a potential therapeutic target for ischemic stroke patients [27].
Figure 2: Proposed mechanisms underlying therapeutic effects of ADMSCs on reducing brain infarct area and improving neurological function [28].
The promotion of angiogenesis has been demonstrated through the transplantation of adipose tissue-derived MSCs in Sprague-Dawley (SD) rats. Following acute ischemic stroke (IS) in adult male SD rats (n = 30), adipose-derived mesenchymal stem cells (ADMSCs) reduced the brain infarction area (BIA) and enhanced neurological function [28]. ADMSCs were cultured two weeks prior to stroke induction in the SD rats. A stroke was induced through the occlusion of the distal left internal carotid artery. The normal controls were ten healthy rats, who did not undergo induction of a stroke. There were two treatment groups of rats with acute IS: group 2 (treated with 1 mL intravenous physiological saline) and group 3 (treated with a dose of 2.0 x 106 intravenous ADMSCs in 0.5 cc culture medium each time). Brain infarct size was measured in five rats from groups 2 and 3. A Corner test was conducted 0, 1, 3, 7, 14, and 21 days post-acute IS induction to test sensorimotor function. CM-DiI, a distinctive fluorescent dye, was added to the culture medium 30 minutes before transplantation to allow visibility of the brain infarct area (BIA) [28].
Figure 3: Comparison of brain infarct area and sensorimotor function in rats treated with (treatment group) and without ADMSCs. (A & B) Location of brain infarct area. (C) The ratio of BIA to total coronal section area. (D) Corner test results on days 0, 3, 7, 14, and 21 following acute IS. (E & F) ADMSCs stained with CM-DiI in the brain infarct area from two rats in the treatment group. Scale bars are representative of 50 µm [28].
The rats treated with ADMSCs presented with a smaller BIA compared to the group without ADMSCs. Furthermore, the treatment group exhibited a reduced ratio of infarct area to total coronal section area. The Corner test, which indicates neurological functional impairment based on the percentage of left turns, demonstrated impairment in both the group with and without ADMSC treatment on day 3. However, by day 21, the treatment group showed significant improvement. The ADMSCs stained with CM-DiI were visible in the brain infarct area within rats in the treatment group, indicating that the ADMSCs migrated to the intended area after IV infusion. These results indicated that ADMSCs have the ability to reduce brain infarct size and to enhance neurological function in rats[28]. Nevertheless, the efficacy of ADMSCs remains contentious. Human umbilical cord blood-derived mesenchymal stem cells (UC-MSCs) are another type of stem cell being investigated for stroke therapy. Because the umbilical cord is deemed medical waste after birth, and the collection process is non-invasive, there is less ethical controversy surrounding the use of UC-MSCs compared to other types of MSCs. Similar to MSCs derived from other sources, UC-MSCs are capable of self-renewal and differentiation. UC-MSCs are specifically targeted due to their immunomodulatory properties, which contributes to an increased interest in their role in regenerative medicine and immunotherapy [29].
In a phase I, open-label trial, there were 10 male adults with acute middle cerebral artery ischemic stroke and aged between 45-79. All the participants were administered a single IV infusion of allogeneic human unrelated donor banked umbilical cord blood (UCB) cells. The infusion was given within 3-10 days after the stroke. Adverse events (AEs) were monitored and used to evaluate the safety of the trial over a 12-monthpost-infusion period. Mild AEs were recorded as grade 1 and 2, moderate AEs were grade 3, severe AEs were grade 4, and grade 5 AEs denoted death occurred. Neurologists monitored physical and neurological status upon screening, starting treatment, and 3 months post-treatment. The mRS was used to determine the level of disability, the NIHSS was used to establish the degree of neurological impairment, and the Barthel Index (BI) monitored the ability of the participant to engage in activities of daily living [30].
The UCB units were matched based on blood type and race and had to satisfy specific criteria, including a targeted cell dose (between 5 x 106 and 5.0 x 107nucleated cell count (TNCC) per kg), viability greater than 85%, negative sterility cultures, and negative maternal infectious disease markers. The allogeneic UCB was administered through a peripheral IV. The infusion was given at most at a rate of 5 ml/kg per hour over the course of 5-30 minutes. The median TNCC infused was 1.68 x 109, the median cell dose infused was 1.54 x 107/kg, and the median viable CD34+ dose infused was 2.03 x 105/kg [30]. The average time of infusion was 7 days post-stroke. mRS is scored from 0 (asymptomatic) to 6 (death). The NIHSS is scored from 0 (normal) to 42 (highly symptomatic), and the BI is scored from 0 (highly dependent) to 100 (independent). Participants showed an average decrease of 1.5 points on the modified Rankin scale, a 6-point decrease on the NIHSS, and a 70-point increase on the BI. All three measures of condition indicated improved outcomes. At 3 months post-infusion, MRIs were performed to assess the evolution of the stroke. The results, in comparison to baseline scans, indicated no significant safety concerns including increases in infarct volume and unexpected bleeding [30].
|
Baseline |
3 months |
mRS |
4 |
2.5 |
NIHSS |
11 |
5 |
BI |
17.5 |
87.5 |
Table 4: The median baseline level and 3-month neurological evaluation results.
Over a 12-month follow-up period, the safety of this trial was assessed by the frequency of study-related AEs and the prevalence of GVHD. The average AE per participant was 10.5 with the total number of reported AEs being 113. Two out of 10 participants experienced a serious AE, and no evidence of GVHD was reported during the follow-up period [30]. The results suggest that following an acute ischemic stroke, an IV infusion of non-HLA matched allogeneic, unrelated donor UCB in adults is achievable and safe without notable adverse effects. Furthermore, 3 months after infusion, all participants demonstrated increased functional outcomes [30].
Neural stem cells are found within a small area between the hilus of the dentate gyrus and the granule cells and are responsible for producing new hippocampal neurons [31]. Preclinically and clinically neural stem cells show promise in the functional recovery of stroke patients when used in IC transplantations. Neural stem cells play a role in the formation of new neurons and the enhancement of neuroregeneration [32]. Transplantation of hNSCs in rat ischemic models shows evidence of differentiation into astrocytes, neurons, and oligodendrocytes [33]. The primary sources that NSCs are extracted from are the neuroectoderm in fetal tissue and the subventricular zone (SVZ) and subgranular zone (SGZ) in adults. Embryonic stem cells (ESCs) and induced pluripotent stem cells (iPSCs) are additional sources of NSC derivation. NSCs are targeted for stroke therapy because of their ability to relocate to places of injury and neurodegeneration within the central nervous system [33]. Direct transplantation of human NSCs into the SVZ after induction of middle cerebral artery occlusion (MCAo) has shown to be safe and to improve neurogenesis. An intraluminal thread occlusion of the left middle cerebral artery was performed to induce a transient focal cerebral ischemic stroke in male SD rats. The occlusion was induced for 2 hours before reperfusion [34]. 5-Bromo-2’-deoxyridine (BrdU) was intraperitoneally injected into all rats (n = 14) in order to expose cell mitosis, which is indirectly indicative of cell proliferation. After 24 hours, following hNSC transplantation, all rats received daily injections of BrdU (50 mg/kg) in saline over the course of 7 days. Untreated rats were infused with phosphate-buffered saline (PBS). Testing of neurological status was performed prior to cerebral ischemia and then on days 1, 7, and 14. The modified Neurological Severity Score (mNSS) was used to test motor, sensory, and reflex status. The results were scored from 0 (normal score) to 20 (maximum deficit score) [34].
hNSCs demonstrated the ability to migrate in the direction of the ischemic striatum and to differentiate within the ischemic lesion. Furthermore, the hNSCs showed improved neurological function in comparison to rats injected with PBS, which was indicated by lower mNSS scores 14 days after transplantation. The brain infarct volume was also lower in rats infused with hNSCs than in rats injected with PBS [34].
Figure 4: Results of hNSC transplantation on neurological function and brain infarct volume in ischemic rats. (A) mNSS results on days 1, 7, and 14 after intraventricular transplantation of hNSCs following MCAo. (B) Infarct volume on day 14 after MCAo in rats treated with PBS and transplanted with hNSCs. (C) Quantification of infarct volume demonstrated in B. A two-sample t-test was used to analyze the data, which was expressed as the mean ± SD (n = 7)[34].
The study also demonstrated an increase in endogenous cell proliferation in ischemic rats transplanted with hNSCs, which was indicated by an increase in proliferating cell nuclear antigens (Pcna). Transplantation of hNSCs also improved the differentiation of endogenous NSCs. Differentiation and expansion were supported by an increased presence of BrdU-positive cells in treated rats compared to untreated rats. Furthermore, focal angiogenesis was improved by the transplantation of hNSCs. The results indicate that NSC therapy is an advantageous method of treatment for ischemic strokes in rats. However, the exact mechanism of recovery after transplantation is indeterminate [34].
Conclusion
The prevalence of stroke in the aging population worldwide renders it necessary to establish a safe and efficacious treatment. Stem cell transplantation shows promising results in the reduction of brain infarct area, decrease in symptoms, increase in independence, and increase in sensorimotor function. Currently, there is no cure for the neurological disease; however, the ability of stem cells to self-renew and differentiate shows promise for the regeneration of damaged cells in the brain. ADMSCs, UC-MSCs, and hNSCs show potential through the ability to reduce brain infarct size, enhance neurological function, differentiate appropriately, and migrate to the site of injury. Mesenchymal stem cells are shown to be safe therapeutic options but have not shown significant patient improvement in clinical trials [32]. Efficacy and safety remain primary concerns for stroke treatment. Although there is support for the use of stem cell therapies in treatment, efficacious treatment has not yet been established. To determine the success of stem cells in stroke treatment, future studies with larger human clinical trials are needed to advance research.
References
- Kuriakose D, Xiao Z (2020) Pathophysiology and treatment of stroke: present status and future perspectives. Int J Mol Sci. 21(20): 7609.
- Murphy SJX, Werring DJ (2020) Stroke: causes and clinical features. Medicine (Abingdon) 48(9): 561-66.
- Yousufuddin M, Young N (2019) Aging and ischemic stroke. Aging (Albany NY) 11(9): 2542-44.
- Ovbiagele B, Nguyen-Huynh MN. (2011) Stroke epidemiology: advancing our understanding of disease mechanism and therapy. Neurotherapeutics 8(3): 319-29.
- Yew KS, Cheng EM. (2015) Diagnosis of acute stroke. Am Fam Physician 91(8):528-36.
- Iwasawa E, Ichijo M, Ishibashi S, Yokota T (2016). The development of collateral circulation and therapeutic prospects in ischemic stroke. Neural Regen Res. 11(3): 368-71.
- Kawabori M, Shichinohe H, Kuroda S, and Houkin K (2020) Clinical trials of stem cell therapy for cerebral ischemic stroke. Int J Mol Sci 21(19): 7380.
- Stonesifer C, Corey S, Ghanekar S, Diamandis Z, Acosta S, et al. (2017) Stem cell therapy for abrogating stroke-induced neuroinflammation and relevant secondary cell death mechanisms. Prog Neurobiol 158:94-31.
- Adams HP, Bendixon BH, Kappelle LJ, Biller J, Love B, et al. (1993) Classification of subtype of acute ischemic stroke. Stroke 24(1):35-41.
- Ay H, Benner T, Arsava EM, Furie KL, Singhal AB, et al. (2007) A computerized algorithm for etiologic classification of ischemic stroke: the causative classification of stroke system. Stroke 38(11): 2979-84.
- Gravanis I, Tsirka SE. (2008) tPA as a therapeutic target in stroke. Expert Opin Ther Targets 12(2): 159-70.
- Saver JL (2005) Time is brain—Quantified. Aha Journals 37(1): 263-66.
- Banks JL, Marotta CA. (2007) Outcomes validity and reliability of the modified rankin scale: implications for stroke clinical trials. Stroke 38(3): 1091-96.
- Chalos V, van der Ende NAM, Lingsma HF, Mulder MJHL, Venema E, et al. (2020) National institutes of health stroke scale. Stroke 51(1): 282-90.
- Hasan TF, Rabinstein AA, Middlebrooks EH, Haranhalli N, Silliman, SL, et al. (2018) Diagnosis and management of acute ischemic stroke. Mayo Clin Proc. 93(4):523-38.
- Lalu MM, Montroy J, Dowlatshashi D, Hutton B, Juneau P, et al. (2019) From the lab to patients: a systematic review and meta-analysis of mesenchymal stem cell therapy for stroke. Transl Stroke Res 11(3): 345-64.
- McCarthy DJ, Diaz A, Sheinberg DL, Snelling B, Luther EM, et al. (2019) Long-term outcomes of mechanical thrombectomy for stroke: a meta-analysis. Scientific World Journal. 7403104.
- Oliveira AJF, Viana SMN, Santos AS (2022). Mechanical thrombectomy for acute ischemic stroke: systematic review and meta-analysis. Einstein (sao Paulo) 20:eRW6642.
- Li W, Shi L, Hu B, Hong Y, Zhang H, et al. (2021) Mesenchymal stem cell-based therapy for stroke: current understandings and challenges. Front Cell Neurosci. 15: 628940.
- Biehl JK, Russell B. (2009) Introduction to stem cell therapy. J Cardiovasc Nurs 24(2): 98-105.
- Borlongan CV. (2019) Concise review: stem cell therapy for stroke patients: are we there yet? Stem Cells Transl Med 8(9): 983-988.
- Ejma M, Madetko N, Brzecka A, Alster P, Budrewicz S, et al. (2022) The role of stem cells in the therapy of stroke. Curr Neuropharmacol. 20(3): 630-647.
- Zhang Y, Dong N, Hong H, Qi J, Zhang S, et al. (2022) Mesenchymal stem cells: therapeutic mechanisms for stroke. Int J Mol Sci 23(5): 2550.
- Mu JD, Ma LX, Zhang Z, Qian X, Zhang QY, et al (2023) The factors affecting neurogenesis after stroke and role of acupuncture. Front. Neurol. 14:1082625.
- Diez-Tejedor E, Gutierrez-Fernandez M, Martinez-Sanchez P, Rodriguez-Frutos B, Ruiz-Ares G, et al. (2014) Reparative therapy for acute ischemic stroke with allogeneic mesenchymal stem cells from adipose tissue: a safety assessment: a phase II randomized, double-blind, placebo-controlled, single-center, pilot clinical trial. J Stroke Cerebrovasc 23(10) 2694-00.
- Tsuji W, Rubin JP, Marra KG. (2014) Adipose-derived stem cells: implications in tissue regeneration. World J Stem Cells 6(3): 312-21.
- Rautiainen S, Laaksonen T, Koivuniemi R (2021) Angiogenic effects and crosstalk of adipose-derived mesenchymal stem/stromal cells and their extracellular vesicles with endothelial cells. Int J Mol Sci 22(19):10890.
- Leu S, Lin YC, Yuen CM, Yen CH, Kao YH, et al. (2010) Adipose-derived mesenchymal stem cells markedly attenuate brain infarct size and improve neurological function in rats. J Transl Med 8:63.
- Nagamura-Inoue T, He H. (2014) Umbilical cord-derived mesenchymal stem cells: their advantages and potential clinical utility. World J Stem Cells 6(2): 195-2.
- Laskowitz DT, Bennett ER, Durham RJ, Volpi JJ, Wiese JR, et al. (2018) Allogeneic umbilical cord blood infusion for adults with ischemic stroke: clinical outcomes from a phase I safety study. Stem Cells Transl Med 7(7): 521-29.
- Lazutkin A, Podgorny O, Enikolopov G. (2020) Modes of division and differentiation of neural stem cells. Behav Brain Res 374: 112118.
- Chrostek MR, Fellows EG, Crane AT, Grande AW, Low WC. (2019) Efficacy of stem cell-based therapies for stroke. Brain Res 1722: 146362.
- Daadi MM, Li Z, Arac A, Grueter BA, Sofilos M, et al. (2009) Molecular and magnetic resonance imaging of human embryonic stem cell-derived neural stem cell grafts in ischemic rat brain. Mol Ther 17(7): 1282-1291.
- Ryu S, Lee SH, Kim SU, Yoon BW. (2016) Human neural stem cells promote proliferation of endogenous neural stem cells and enhance angiogenesis in ischemic rat brain. Neural Regen Res. 11(2): 298-4.