The Effect of Stem Cells on Bronchopulmonary Dysplasia
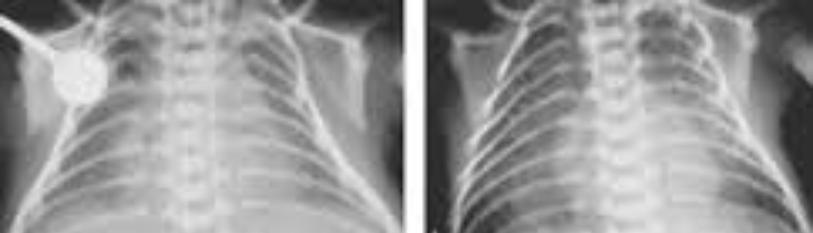
Keeghan Andrews and Vincent S. Gallicchio*
Department of Biological Sciences, College of Science, Clemson University, Clemson, SC 29636
*Corresponding author: Vincent S. Gallicchio, Department of Biological Sciences, College of Science, Clemson University, Clemson, SC 29636
Citation: Andrews K , Gallicchio VS. (2022) The Effect of Stem Cells on Bronchopulmonary Dysplasia. J Stem Cell Res. 3(1):1-20.
Received: February 04, 2022 | Published: February 22, 2022
Copyright© 2022 genesis pub by Andrews K, et al. CC BY-NC-ND 4.0 DEED. This is an open-access article distributed under the terms of the Creative Commons Attribution-Non Commercial-No Derivatives 4.0 International License., This allows others distribute, remix, tweak, and build upon the work, even commercially, as long as they credit the authors for the original creation.
DOI: https://doi.org/10.52793/JSCR.2022.3(1)-28
Abstract
Bronchopulmonary dysplasia (BPD) affects over 15,000 infant births per year in the United States and is one of the major causes of mortality in preterm infants. BPD is one of the most common long-term adverse effects from premature infant birth. Unlike other medical complications related to premature infant birth, the incidence of BPD is not declining. Approximately 15 million infants are born prematurely each year. One million infants worldwide are reported to die each year as a result of medical complications due to premature birth. Therapeutic interventions for BPD include: maternal progesterone, antenatal steroids, surgical cerclage, decreased ventilation levels, caffeine/methylxanthines, diuretics, bronchodilators, postnatal steroids, vitamin A, and inhaled nitric oxide. These interventions have demonstrated limited effectiveness. Mesenchymal stem cells (MSCs) are the preferred source for therapy for infants with BPD as they differentiate into various cell types including: muscles, bones, fat, and cartilage. Stem cell transplantation has shown to improve lung function and repair, decrease pulmonary hypertension, decrease risk for neurodevelopmental disorders, decrease BPD severity, and increase survival rates. Researchers believe the use of stem cells may offer alternative options for patients with BPD to improve lung function and decrease complications related to premature birth.
Keywords
Bronchopulmonary dysplasia; Stem cells; Treatment
Introduction
Bronchopulmonary dysplasia (BPD) affects over 15,000 infant births per year in the United States and is one of the major causes of mortality in preterm infants [1-5]. BPD is one of the most common long-term adverse effects from premature infant delivery. Infants born with very low birth weight (VLBW) develop BPD at a rate of 30% - 40%, while infants born with extremely low birth weight (ELBW) develop BPD at a rate of 54.1% [6-11]. Many infants with BPD are readmitted to the hospital for lower respiratory infections within the first two years of life. Single hospital readmission rates for infants with BPD are reported to be 73% and multiple readmission rates (3 or more hospital readmissions) are reported to be 27% [3,12,13]. The overall mortality rate for infants with BPD is 15% and increases to 41% for infants with severe BPD [12,14]. Unlike other medical complications related to premature infant birth, the incidence of BPD is not declining [15].
The World Health Organization (WHO) defines preterm birth as an infant born before 37 weeks of pregnancy. Premature birth is a medically complex diagnosis and a substantial global public health problem. Approximately 15 million infants are born prematurely each year, accounting for 11% of all live births worldwide. The incidence of premature birth for infants in North America is at 12.5% and increasing. Severe medical complications related premature birth include: periventricular leukomalacia (PVL), necrotizing enterocolitis (NEC), retinopathy of prematurity (ROP), hypoxic-ischemic encephalopathy (HIE), and bronchopulmonary dysplasia (BPD) [16-19]. One million infants worldwide are reported to die each year as a result of medical complications due to premature birth [5,15-17,20]. Survival rates for infants improves as gestational age increases. Premature infants born at 28 weeks gestation had a 92% chance of survival while infants born at 22 weeks gestation had a 6% chance of survival [21].
Northway first described BPD as a syndrome of chronic disease occurring in preterm infants that are treated for respiratory distress syndrome with mechanical ventilation and supplemental oxygen [4,17,22-26]. National Institute of Child Health and Human Development (NICHD) defined BPD as a condition in which the infant requires treatment with supplemental oxygen for more than 28 days and additional details are outlined for infants at 36 weeks post-menstrual age indicating severity based on the level of respiratory support required by the infant [9,27]. During the early stages of BPD, patients usually require ventilator or supplemental oxygen support. Most patients gradually withdraw from the ventilator or stop supplemental oxygen treatment based upon BPD level of severity and medical complications [12].
NIDCD BPD Severity Scale
Improvements in perinatal medicine have led to an increased rate of survival for extremely premature and low birth weight infants. Improved survival rates for premature infants has led to increased rates of BPD. Patients with BPD are less mature and have lower birthweights than described over 50 years ago [5,14,15,21,22,29-31]. Infants are now surviving at earlier gestational ages. Medical care provided in the Neonatal Intensive Care Unit (NICU) for patients with BPD has adapted to meet the changing needs of these medically complex patients [24] (Table 1).
|
|
mild BPD |
breathing room air |
moderate BPD |
need for <30% oxygen |
severe BPD |
need for > 30% oxygen and/or positive pressure ventilation (PPV) or continuous pressure airway pressure (CPAP) |
Table 1: Lists severity levels of BPD based on NIDCD criteria [4,28].
Prematurity Levels
Lung development includes several stages: embryonic, pseudoglandular, canalicular, saccular, and alveolar. Preterm infant lungs must demonstrate continued alveolar growth and differentiation after birth. Infant preterm birth can lead to impaired alveolarization and abnormal lung growth, even without introducing mechanical ventilation or supplemental oxygen (Table 2). An infant breathing room air results in oxygen exposure that is at least five times higher than oxygen concentration of the amniotic fluid the infant encountered in utero. Infants born before 30 weeks gestation are usually exposed to supplemental oxygen during the late canalicular or saccular lung development stages. Additional perinatal risk factors are show in (Figure 2) [2,3,23,24,27,33-35].
late preterm |
34-36 weeks gestation |
moderate preterm |
32-34 weeks gestation |
very preterm |
<32 weeks gestation |
extremely preterm |
<25 weeks gestation |
Table 2: Lists prematurity severity levels based upon weeks gestation [32].
Anatomical Stages of Infant Lung Development
Figure 1: Outlines anatomical infant lung development stages: embryonic, pseudoglandular, canalicular, saccular, and alveolar [36].
Figure 2: Outlines multifactorial etiology of BPD: antenatal and postnatal factors that contribute to BPD [27].
Advances in perinatal medical care over the past several years have improved survival rates for preterm infants from 34 weeks gestation to 24 weeks gestation. Preterm infants are now born with more immature lungs and the pathology of BPD has changed. Today, BPD is characterized by impaired alveolar development, decreased pulmonary microvascular growth, and less pulmonary inflammation compared to the original characteristics of BPD several years ago [3,4,9,17,24,28,31,37].
Preterm infants born between 24 and 28 weeks have a higher risk of developing BPD due to interruption of the canalicular and saccular stages of lung development. Differentiation of epithelial cells begins during canalicular stage. This stage is important for surfactant phospholipid and protein development that support immunological protection and lung stability. During the saccular stage, the interstitial space of the saccular walls decreases. Secondary crests are formed and cylindrical saccules develop double capillary layer. Alveolar stage continues after birth and through childhood. During alveolar stage, secondary crest is extended and thinned which leads to fusion of capillaries to help form alveoli in the lungs [6,11,24,28,34,38].
Pathogenesis of BPD
Risk factors for BPD include: maternal smoking and hypertension, low birth weight, premature birth, intrauterine growth restriction (IUGR), genetic factors including SPOK2 gene, prenatal and postnatal infections, mechanical ventilation, and poor infant nutrition after birth [3,6,11,25,39].
Figure 3: Outlines the pathogenesis of BPD including distortion of alveolar, mesenchymal, and vascular development primarily in saccular stage [11].
BPD is a leading cause of mortality and long-term respiratory complications that can continue into adulthood including: airway hyperreactivity, poor lung function, asthma, wheezing, chronic obstructive pulmonary disease, and early onset emphysema. Premature infants with BPD are also at increased risk for neurodevelopmental complications including developmental delay and cerebral palsy [1-3,14,20,25,29,40-45].
Northway et al., studied the pulmonary function of 52 adolescents and young adults with BPD and compared them to 53 healthy human control subjects born full-term. The authors reported that 68% of the subjects with a history of BPD demonstrated airway problems including airway obstruction, lower lung capacity, and lower expiratory volume as compared to 17% of healthy control subjects. Additionally, subjects with BPD were physically shorter in height and weighed less than the normal controls. Five of the subjects with BPD were diagnosed with cerebral palsy [3,46].
Robin et al., examined lung function in infants with BPD through spirometry measurements. Lung function for 28 preterm infants and 41 healthy full-term infants were studied. Results indicated patients with BPD demonstrated pulmonary function abnormalities leading to mild to moderate obstruction of airflow and trapping of air [3,47].
Fakhourg, et al., evaluated lung function of 44 children with BPD during the first 3 years of life. Data was gathered for each subject at 6, 12, and 24 months of age. Results indicated children with moderate to severe BPD showed abnormalities with airflow limitation characterized by low partial expiratory airflow. This lung function impairment did not improve over time throughout the duration of the study [48,49].
Aukland et al., used high resolution computed tomography (CT) to study the structural lung injury for a group of 74 subjects age 10 to 18 years with a history of BPD. Pathological findings were detected in 86% of adolescents and children including linear/triangular opacities [3,50].
Karila et al., evaluated pulmonary function during exercise activities for 20 subjects with a history of BPD. The subjects of this study were 7-14 years of age and compared with age matched normal peers without a history of BPD. During this study, 60% of the subjects with BPD demonstrated hyperoxemia, alveolar hypoventilation, and lower tidal lung volume during exercise activities as compared to no reported lung function issues for age matched peers. Results of this study indicate subjects with BPD may have extended issues related to lung function that can affect aerobic exercise activities into childhood and adolescence [3,51].
Wong et al., investigated long term lung functional outcomes and medical complications for adult subjects with a history of BPD. Twenty-one subjects were included in this study with an age range of 17-33 years. Results of this study revealed 71% of the subjects had persistent respiratory symptoms including decreased expiratory volume and decreased lung vital capacity. Emphysema was found to be present in 84% of subjects [5,52].
BPD Treatments
Several antenatal and postnatal treatments are available to treat BPD: maternal progesterone, antenatal steroids, surgical cerclage, decreased ventilation levels, caffeine/methylxanthines, diuretics, bronchodilators, postnatal steroids, vitamin A, and inhaled nitric oxide [3,6,22,26,28].
Prevention of premature birth remains the most effective course of action to reduce the risk of BPD. Interventions to prevent premature birth include: maternal progesterone supplementation, prevention of pregnant women exposure to cigarette smoke, surgical cerclage procedure to close the cervix, and avoidance of fertility treatments that may result in multiple fetuses. Maternal progesterone has been shown to reduce the risk of preterm birth at less than 34 weeks. Progesterone supplementation from 16 to 24 weeks gestation is recommended by the American College of Obstetricians and Gynecologists to decrease preterm birth. Administration of antenatal steroids for women at risk for premature birth has shown decreased severity of acute respiratory disease and mortality for the infants [3,26].
Mechanical ventilation is required to maintain adequate infant lung volumes, but may also lead to negative effects. Ventilation can damage the epithelium and cause inflammation of the lungs. Extended mechanical ventilation is associated with an increase of chronic lung disease, sepsis, and neurodevelopmental impairment for the infant. Adjusting mechanical ventilation pressure levels and avoiding endotracheal tube intubation can promote improved outcomes for infants. Utilization of nasal continuous positive airway pressure (NCPAP) or high flow nasal cannulas (HFNCs) for infants had a reported 95% beneficial impact on preventing BPD for infants who tolerated these procedures [3,23].
Caffeine has been shown to reduce apnea and consequently the need for ventilation leading to decreased risk for BPD. Caffeine for Apnea for Prematurity Trial showed that caffeine reduced incidence of BPD and neuro-disability at 18 months of age for infants who received caffeine after birth [4,28]. Caffeine improved tidal lung volume and respiratory drive and reduced lung resistance. This led to more stabilized chest wall and improved functional residual capacity to allow for earlier extubation. Side effects of caffeine including temporary weight loss and tachycardia are mild to low risk. Caffeine doses are recommended early within the first 2 days of life, but earlier caffeine doses may be associated with an increased risk for necrotizing enterocolitis (NEC) [3,31].
Diuretics may be used to help reduce fluid overload and pulmonary edema for premature infants. Premature infants often receive large volumes of intravenous fluids to provide adequate hydration and nutrition [3,26]. Furosemide is a commonly used diuretic that can decrease interstitial edema and pulmonary resistance and increase lymphatic flow. Alternate day dosages of diuretics are recommended to improve clinical respiratory status, oxygenation, and support weaning from mechanical ventilation for premature infants. Long term benefits of diuretics have not been established for infants with BPD. A few side effects of diuretics may include: ototoxicity, electrolyte disturbances, and azotemia [4,14].
Inhaled bronchodilators such as Albuterol may be recommended for infants with BPD. Bronchodilators aim to improve lung mechanics following bronchospasm [26]. Bronchodilators focus on smooth muscle relaxation that can lead to improved pulmonary resistance and lung compliance. Short term improvement benefits have been shown with bronchodilators, but long term effects have not been established. Additionally, tolerance may develop with prolonged use of bronchodilators [4]. Infants with BPD may also have other airway abnormalities including tracheomalacia and bronchomalacia. For infants with tracheomalacia, use of bronchodilators can worsen wheezing by causing smooth muscle relaxation in an airway that is already floppy [14].
Corticosteroids and surfactant administered soon after birth can lead to decreased risk for BPD. Corticosteroids can stimulate surfactant phospholipids production by alveolar type II cells, enhance surfactant associated proteins, and accelerate overall maturation of the structure of the lungs. Combination of corticosteroids and surfactant together can improve gas exchange, mature development of the lung, and reduce lung inflammation for premature infants [3,31]. Corticosteroids and surfactant can support early extubation for infants with BPD. Long term effects of surfactant were reported. At one year of age, infants who received surfactant shortly after birth required fewer hospital admissions for respiratory complications than infants who did not receive surfactant [9,20]. Possible corticosteroid side effects may include: hyperglycemia, hypertrophic cardiomyopathy, retinopathy of prematurity, hypertension, and cerebral palsy [3,4,14,25].
Vitamin A is critical for normal development and tissue repair of the infant respiratory tract and lungs. Preterm infants typically have low vitamin A levels at birth. Infants that received supplemental vitamin A after birth showed lower risk for BPD. This effect was only observed for premature infants weighing <1000 [4,28]. Vitamin A is not reported to reduce mortality, reduce length of ventilation time, reduce hospital length of stay, or improve neurodevelopmental outcomes at age 18-22 months. Possible side effects of vitamin A supplements may include painful intramuscular injections 3 times per week to total up to 12 doses and an increased sepsis risk [3,20].
Inhaled nitric oxide has demonstrated minimal benefit for decreased pulmonary vascular resistance and increased oxygenation. Clinical use of inhaled nitric oxide lacks support for clinical use. A meta-analysis of studies investigating the use of inhaled nitric oxide found no significant clinical advantages. Routine use of inhaled nitric oxide is not advised by medical experts and the scientific community. Inhaled nitric oxide is reported to have a high cost and is not supported by medical professionals due to lack of clinical evidence-based research [3,48]. One study conducted by Van Meurs et al., reported a higher rate of mortality and intraventricular hemorrhage (IVH) in infants who received inhaled nitric oxide [21].
Pharmacological treatments for BPD
maternal progesterone |
|
caffeine |
|
diuretics |
|
bronchodilators |
|
antenatal steroids |
|
surfactant |
|
vitamin A |
|
inhaled nitric oxide |
|
Discussion
Despite advances in perinatal and neonatal medicine, limited progress has been made in reducing the rate of infants diagnosed with BPD. Mesenchymal stem cells (MSCs) are the current leading cell product under investigation for the prevention of BPD and other medical complications related to infant premature birth [12,35,53,54]. MSCs offer innovative therapy and have the ability to self-renew and differentiate into various cell types including: muscles, bones, fat, and cartilage. MSCs originate from the mesoderm and can be obtained from many sources including: tendon, blood, skin, umbilical cord, bone, adipose tissue, and amnion [22,28,41,55]. Human umbilical cord blood derived mesenchymal stem cells (hUCBMSCs) are an abundant source of MSCs as they do not require an invasive harvesting procedure required for bone marrow or adipose MSCs. MSCs have a good potential to develop into adipocytes, myoblasts, chondrocytes, and osteoblasts. MSCs are able to mitigate lung inflammation, induce vascular and alveolar growth, and inhibit lung fibrosis [2,6,16,22,26,56].
Mesenchymal Stem Cell Function
Initial research supported MSCs showing benefit through a regenerative process homing injured tissues, engrafting, and replacing damaged cells. Newer evidence suggests MSCs offer benefits through secretomes and paracrine action. MSCs exert immunomodulatory, anti-inflammatory, angiogenic, antibacterial, and pro-regenerative effects [16,37,58]. MSCs secrete growth promoting and regeneration stimulating factors including: angiopoietin 1, epidermal growth factor, hepatocyte growth factor, keratinocyte growth factor, and vascular endothelial growth factor alpha, which drive repair and regeneration. MSCs can sense signals in the surrounding damaged tissues, modulate paracrine actions in response, and secrete mediators able to reduce inflammation and repair injured tissues [6,7,18,35,41,59] (Figure 4).
Initial research supported MSCs showing benefit through a regenerative process homing injured tissues, engrafting, and replacing damaged cells. Newer evidence suggests MSCs offer benefits through secretomes and paracrine action. MSCs exert immunomodulatory, anti-inflammatory, angiogenic, antibacterial, and pro-regenerative effects [16,37,58]. MSCs secrete growth promoting and regeneration stimulating factors including: angiopoietin 1, epidermal growth factor, hepatocyte growth factor, keratinocyte growth factor, and vascular endothelial growth factor alpha, which drive repair and regeneration. MSCs can sense signals in the surrounding damaged tissues, modulate paracrine actions in response, and secrete mediators able to reduce inflammation and repair injured tissues [6,7,18,35,41,59].
Figure 4: Outlines the interaction of MSCs with B cells, NK cells, macrophages, monocytes, dendritic cells, neutrophils, and T cells [57].
As MSCs can regenerate and differentiate, a concern was raised whether stem cells could possibly differentiate into malignant tumor cells. The immunosuppressive environment created by MSCs may have the potential to lead to the development and transformation of bone marrow MSCs. Preclinical studies revealed there is no evidence of malignant tumor development or adverse events up to 60 months post MSC treatment. Current research supports the theory that MSCs will not change spontaneously into malignant tumors [6].
Stem Cell Trials in Animals for BPD
A meta-analysis completed by Augustine et al., in 2017 reported the outcomes for rats that received MSCs was better than the control group that did not receive MSCs for intraperitoneal, endotracheal, and intravenous administration of MSCs. Subjects that received MSCs showed improved alveolarization, decreased lung inflammation, and improved pulmonary artery remodeling. Intravenous administration of MSCs showed improved outcomes as compared to intratracheal administration of MSCs. No significant differences were found between low, middle, and high doses of MSCs. The timing of administration of MSC treatments showed no significant difference for clinical outcomes [6,9,60].
Vanhaaftan et al., in 2009 investigated the effect of intratracheal delivery of bone marrow derived mesenchymal stem cells (BMSCs) as a possible treatment to prevent alveolar destruction in BPD. The potential therapeutic effect of BMSCs was evaluated in a hyperoxia-induced model of BPD in newborn rats. Results showed chronic hyperoxia in newborn rats led to loss of lung capillaries, air space enlargement, and decreased resident lung BMSCs. Administration of BMSCs on postnatal day 4 revealed decreased alveolar and lung vascular injury, improved survival and exercise tolerance, and decreased pulmonary hypertension. This study suggested BMSCs may be a therapeutic option with positive outcomes for subjects with BPD [5,6].
Aslam et al., in 2009 studied the effect of BMSCs on BPD utilizing neonatal mice exposed to hyperoxia. Mice were injected intravenously on day 4 with BMSC or BMSC conditioned media and evaluated on day 14 for vascular changes associated with pulmonary hypertension, lung morphometry, and lung cytokine profile. Results indicated injection of BMSCs prevented pulmonary hypertension and reduced alveolar loss and lung inflammation. Injection of BMSC conditioned media prevented vessel remodeling and alveolar injury. This study suggested that BMSCs could offer new therapeutic approaches for lung diseases [61].
Zhang et al., in 2012 evaluated the effects of BMSC in vitro and in vivo in mouse models. Intraperitoneal injection of BMSCs occurred on postnatal day 7. BMSCs migrated toward the injured lung, expressed surfactant protein-C, improved pulmonary architecture, decreased pulmonary fibrosis, and increased the survival rate of mice with BPD. This study supports the use of BMSCs to help regulate inflammation and fibrosis of injured lung tissue after hyperoxia [62].
Hansmann et al., in 2012 designed a study to investigate if MSCs with conditioned media could help reverse hyperoxia induced BPD and pulmonary hypertension. During this study, newborn mice were exposed to hyperoxia for two weeks, treated with one intravenous dose of MSCs with conditioned media, and placed in room air for 2-4 weeks. Histological analysis of lungs was completed at 4 weeks. Pulmonary artery pressure tests, echocardiography, and pulmonary function tests were conducted at 6 weeks. Results of this study revealed subjects that received MSCs with conditioned media demonstrated: reversal of hyperoxia-induced parenchymal fibrosis, normalized lung function, partially reversed alveolar injury, and fully reversed pulmonary hypertension. This study suggests MSCs with conditioned media can support reversal of symptoms associated with hyperoxia-induced BPD [63].
Sutsko et al., in 2013 examined if long-term reparative effects for a single intratracheal dose of MSCs or MSC conditioned medium are comparable in established hyperoxia induced lung injury. In this study, newborn rats exposed to hyperoxia were randomized to receive MSCs, MSC conditioned medium, or placebo. Alveolarization and angiogenesis were evaluated on days 16, 30, and 100. All time periods revealed improvements in alveolar and vascular development and decreased inflammation for rats treated with MSCs and MSC conditioned media compared with the control placebo group. On day 100, improvements were more significant for the MSC group compared to MSC conditioned medium group. Results of this study support the use of MSCs and MSC conditioned medium as a possible treatment for lung injury [6,64].
Pierro et al., in 2013 investigated the effect of human umbilical cord blood derived MSCs (hUCB-MSCs) delivered through intratracheal injection to rat pups exposed to hyperoxia. Rat pups exposed to hyperoxia demonstrated arrest in alveolar growth through loss of lung capillaries and air space enlargement. hUCB-MSCs partially prevented and restored lung function in the rat pups. Although the amount of cell implantation was low, lung repair and function continued to improve over a long period due to the paracrine effects of hUCB-MSCs. Long term outcomes at six months of age showed no adverse events in the stem cell group. Continued improvement in both lung structure and exercise capacity was demonstrated. This study suggests hUCB-MSCs may offer short and long term befits for treatment of lung diseases [6,9,65].
Ahn et al., in 2013 conducted a study that evaluated long term effects and safety of intratracheal transplantation of hUCB-MSCs in rat model of neonatal hyperoxic lung injury. In this study, rat pups were subjected to hyperoxia at birth. The rat pups received intratracheal hUCB-MSCs on day 5. On day 70, the pups were sacrificed and organs including the lung, heart, liver, and spleen were histologically examined. Results revealed no gross or microscopic abnormal findings in rat pups in the liver, heart, or spleen related to transplantation of MSCs. This study suggests therapeutic use of hUCB-MSCs does not lead to long term adverse side effects [6,66].
Wecht et al., in 2016 studied the mechanism of repair used by BMSCs in response to lung injury utilizing mouse subjects. BMSCs were administered intratracheally to mice with and without myelosuppression 6 hours after exposure to bleomycin. Before administration of BMSCs, 1/3 of myelosuppressed mice lived 14 days after exposure to bleomycin. All mice without myelosuppression lived 14 days. When BMSCs were administered, both normal and myelosuppressed mice all survived 14 days after bleomycin exposure. BMSCs migrated to the source of injury, adopted parenchymal cell phenotypes, decreased inflammation, and limited fibrosis in lung tissue. After BMSC administration, immune related cytokines returned to normal levels and promoted a more suitable environment for repair. Results of this study indicate BMSCs can play a role in protection and repair of lung after bleomycin injury [6,67].
Chen et al., in 2017 evaluated the effect of a surfactant combined with human MSCs on lung function. Human MSCs and surfactant treated MSCs were injected intratracheally into newborn rats on postnatal day 5 and study groups were evaluated on postnatal day 14. Surfactant treated MSCs showed reduced viability after exposure to surfactant. Surface marker expression marker profile evaluation indicated surfactant did not alter the phenotype of MSCs during this study. Rats who received surfactant or surfactant treated MSCs exhibited higher rate of survival than those who did not receive treatment. Differences in survival rate between MSC and surfactant treated MSC was not statistically significant. Results of this study indicated combination therapy involving a surfactant and MSCs together does not yield additional positive effects on hyperoxia-induced lung injury [55].
Animal Clinical Trials for Treatment of BPD
Author (year) |
Dose (per animal), time (hours), method |
Outcomes |
van Haaften (2009) |
(1) 1 × 105 BMSC, P4, IT |
alveolarization, lung angiogenesis, pulmonary hypertension, exercise capacity, survival rate |
(2) BMSC 1 × 105, P14, IT |
||
Aslam (2009) |
(1) 5 × 104 cells P4, IV |
alveolarization, lung inflammation, pulmonary hypertension, vascular injury |
(2) BMSC-CM 50 μl, P4, IV |
||
Zhang (2012) |
1 × 105 cells, P10, IV |
lung inflammation, alveolarization, tissue cytokine |
Sutsko (2013) |
(1) 2 × 106 cells, P9, IT |
alveolarization, lung angiogenesis & inflammation |
(2) 0.05 ml MSC-CM, IT |
||
Pierro (2013) |
(1) 3 × 105 MSCs, P4, IT |
alveolarization, pulmonary remodeling, pulmonary hypertension, lung angiogenesis, function, exercise capacity |
(2) 6 × 105 MSCs, P14, IT |
||
(3) 7 μl/g CdM, P4-P21, IP |
||
(4) 7 μl/g CdM, P14-P28, IP |
||
Ahn (2013) |
5 × 105 cells, P5, IT |
alveolarization, lung inflammation, angiogenesis, safety, weight, survival rate |
Ahn (2015) |
(1) hUB-MSC 5 × 105 cells |
alveolarization, lung inflammation, angiogenesis |
(2) AT MSC 5 × 105 cells |
||
(3) hUB-MNC 5 × 105 cells; P5, IT |
Table 4: Lists current clinical research trials investigating the use of MSC to treat BPD in animal models [6].
Stem Cell Trials in Humans for BPD
Chang et al., in 2014 evaluated the safety of hUCMSCs in preterm infants at risk for BPD. Three patients were administered an intratracheal low dose of hUCMSCs and 6 additional patients were given a high dose of hUCMSCs 10 days after birth. Oxygen therapy after discharge was not required for infants who received hUCMSCs, while 22% of the control group that did not receive hUCMSCs required oxygen therapy after discharge. BPD severity was lower for patients that received hUCMSCs and adverse outcome results did not differ between the control group and the group of patients that received hUCMSCs. Results of this study support the use of hUCMSCs in preterm infants as a safe treatment option to improve lung function. An additional phase II study with an increased number of subjects was recommended [6,56].
Ahn et al., in 2017 researched long-term safety and outcomes of MSC administration for 9 premature infants enrolled in a previous phase I clinical trial for up to 2 years of age. Several outcomes including: adverse events, respiratory issues, somatic growth, and neurodevelopmental performance were evaluated at consecutive visits scheduled at 4-6 months, 8-12 months, and 18-24 months. Results indicated 1 of 9 infants died of Enterobacter cloacae sepsis at 6 months of age in the MSC group and the remaining 8 infants survived without having any MSC related adverse outcomes. All infants in the MSC group were discharged home without supplemental oxygen requirements, compared to 22% in the control group. Average rate for hospital readmissions due to respiratory issues was 1.4 readmissions per patient. Average body weight for the MSC group was significantly higher than the control group at 18-24 months of age. In the MSC group, no infants were diagnosed with blindness, cerebral palsy, or developmental delay as compared to 1 infant diagnosed with cerebral palsy and 1 infant with developmental delay in the control group. Results of this study suggested use of MSCs in preterm infants appears to be safe without adverse effects on growth, respiratory status, and neurodevelopmental skills at 2 years of age [29].
Alvarez–Fuente et al., in 2018 investigated 2 infants with severe BPD who received repeated intravenous doses of allogeneic bone marrow-derived MSCs. Temporal profile of serum, tracheal cytokines, and growth factors were analyzed. Patient safety, tolerability and clinical response were also monitored. Results from this limited study suggested that administration of repeated intravenous doses of MSCs was safe and led to decreased pro-inflammatory molecules and lung injury biomarkers. Both patients did not survive the disease as they were both at very advanced stages of BPD with severe lung fibrosis. This study supports the use of MSCs for patients with BPD, but recommends MSCs administration during the early stages of BPD disease [58].
Lim et al., in 2018 designed a study to evaluate the use of human amnion epithelial cells (hAECs), stem-like cells derived from placental membranes, in infants with BPD to assess the safety of these cells. Six premature infants with BPD received intravenous infusion of hAECs. This study focused on safety including: local site reaction, infection, anaphylaxis, features of rejection, and tumor formation. Results of this study indicated hAECs were well tolerated. The first baby demonstrated transient cardiorespiratory compromise during cell administration that was consistent with a pulmonary embolic event. Changes were implemented to cell administration methods including: reducing cell concentration, addition of inline filter, and reducing rate of cell infusion. No additional negative adverse events were reported for the other 5 infants. Results of this study suggest hAECs may be safely infused into infants with BPD. Additional clinical trials with larger sample size of patients was recommended [68].
Powell et al., in 2019 evaluated the safety of hUCMSCs for infants at risk for BPD. Twelve extremely low birth weight infants <28 weeks gestation and <1000 grams at birth received an intratracheal single dose of hUSMSCs at 10 days of life. Six infants received a low dose of hUSCMSCs and 6 infants received a high dose of hUSCMSCs. Results of the study revealed no significant clinical differences between low dose and high dose groups. The treatment was tolerated and appeared to be safe. Additional research was recommended with a larger randomized controlled blinded study [2,6].
Future and Ongoing Stem Cell Trials in Humans for BPD
Park et al., in 2014 designed a study to evaluate long term outcomes of hUCMSCs intervention on BPD. This study will evaluate and monitor long term outcomes of therapy using neurological development tests and growth measures and compare hUCMSCs intervention with a placebo group. Sixty-two premature infants with BPD have been enrolled in this study. Each infant received a single intratracheal administration of hUCMSCs. Follow up assessments will be completed at: 6 months, 12 months, 18 months, 24 months, 36 months, 48 months, and 60 months. Several medical and clinical factors will be assessed and monitored: medical treatments including use of oxygen, steroids, bronchodilators, admissions to emergency room due to respiratory issues, survival rate, growth measured by percentile charts, neurological development measured by the Bayley Scale of Infant and Toddler Development, motor development measured by Gross Motor Function Classification System for Cerebral Palsy, incidence of deafness or blindness, number of adverse events, significant changes in physical exam, and brain MRI results. Patient data collection dates: January 2014 through March 2020. Clinical Trials.gov ID NCT01897987 [43,53,54,69].
Park et. al., in 2020 initiated a study that will attempt to establish safety parameters, maximum administration dosages and feasibility of hUCMSCs delivered intravenously to preterm infants at risk of developing BPD. This will be a Phase 1, open-label, single center, dose-escalating trial using a 3+3+3 design. The first three patients will receive 1 million cells/kg of body weight, the next three patients will receive 3 million cells/kg of body weight, and the final three patients will receive 10 million cells/kg of body weight. A total of 60 preterm infants at risk for developing BPD will be enrolled in this study rotating through the 3+3+3 trial design. Outcome measures include: incidence and severity of BPD, rate of death, blood culture confirmed sepsis, patent ductus arteriosus (PDA), necrotizing enterocolitis (NEC), retinopathy of prematurity (ROP), severe intraventricular hemorrhage (IVH) ≥ grade 3, periventricular leukomalacia (PVL), pulmonary hypertension, duration of ventilator support, duration of supplemental oxygen, postnatal steroids, and neurodevelopmental scores evaluated by Bayley Scale of Infant and Toddler Development. Study start date: July 2019 and expected end date: June 2027. Clinical Trials.gov ID: NCT04003857 [6].
Current Clinical Human Trials for MSC Treatment for Infants with BPD
NCT number |
Status |
Enrollment |
Cell type |
Start date |
Completion date |
NCT03601416 |
Not yet recruiting |
57 |
MSC |
43647 |
44561 |
NCT03378063 |
Recruiting |
100 |
hMSC |
43040 |
44925 |
NCT03631420 |
Recruiting |
9 |
hUC-MSC |
43399 |
44865 |
NCT04255147 |
Not yet recruiting |
9 |
hUCT-MSC |
44582 |
13119 |
NCT02443961 |
Recruiting |
10 |
MSC |
43557 |
44676 |
NCT03774537 |
Recruiting |
20 |
hUC-MSC |
43525 |
44561 |
NCT01632475 |
Active, not recruiting |
9 |
hUC-MSC |
44815 |
44830 |
NCT04003857 |
Recruiting |
60 |
hUC-MSC |
43651 |
46568 |
NCT03558334 |
Recruiting |
12 |
hMSC |
43279 |
44742 |
Conclusion
Current medical intervention treatments for human infants with BPD include: maternal progesterone, antenatal steroids, surgical cerclage, decreased ventilation levels, caffeine/methylxanthines, diuretics, bronchodilators, postnatal steroids, vitamin A, and inhaled nitric oxide. These interventions have demonstrated limited effectiveness. MSC transplantation has been introduced in both animal and human subjects for treatment of BPD. Researchers have reported stem cell transplantation for BPD in animal and human models has improved lung function and repair, decreased pulmonary hypertension, decreased risk for neurodevelopmental disorders, decreased BPD severity, and increased survival rates.
Several current research studies are currently in process for patients with BPD. These studies are investigating safety, efficacy, and long-term outcomes for the use of MSCs to treat BPD extending up to 5 years post MSC transplantation procedure.
Researchers believe the use of stem cells may offer alternative options for patients with BPD to improve lung function and decrease complications related to premature birth. Additional research trials are needed to further investigate the functional effects and efficacy for stem cell transplantation for infants with BPD.
References
- Suzuki T, Sato Y, Yamamoto H, Kato T, Kitase Y, et al. (2020 Mesenchymal stem/stromal cells stably transduced with an inhibitor of CC chemokine ligand 2 ameliorate bronchopulmonary dysplasia and pulmonary hypertension. Cytotherapy. 22(4):180-192.
- Powell SB, Silvestri JM. (2019) Safety of Intratracheal Administration of Human Umbilical Cord Blood Derived Mesenchymal Stromal Cells in Extremely Low Birth Weight Preterm Infants. J Pediatr.210:209-13.e2.
- Principi N, Di Pietro GM, Esposito S. (2018). Bronchopulmonary dysplasia: clinical aspects and preventive and therapeutic strategies. J Transl Med. 16(1):36.
- Iyengar A, Davis JM. (2015) Drug therapy for the prevention and treatment of bronchopulmonary dysplasia. Front Pharmacol. 6:12.
- van Haaften T, Byrne R, Bonnet S, Rochefort GY, Akabutu J, et al. (2009) Airway delivery of mesenchymal stem cells prevents arrested alveolar growth in neonatal lung injury in rats. Am J Respir Crit Care Med. 180(11):1131-42.
- Tong Y, Zuo J, Yue D. (2021) Application Prospects of Mesenchymal Stem Cell Therapy for Bronchopulmonary Dysplasia and the Challenges Encountered. Biomed Res Int.2021:9983664
- Lesage F, Thébaud B. (2018).Nanotherapies for micropreemies: Stem cells and the secretome in bronchopulmonary dysplasia. Semin Perinatol. 42(7):453-58.
- Simones AA, Beisang DJ, Panoskaltsis-Mortari A, Roberts KD. (2018) Mesenchymal stem cells in the pathogenesis and treatment of bronchopulmonary dysplasia: a clinical review. Pediatric Res. 83(1-2)308-17.
- Williams E, Greenough A. (2019) Advances in treating bronchopulmonary dysplasia. Expert Rev Respir Med. 13(8):727-35.
- Mueller M, Kramer BW. (2017) Stem cells and Bronchopulmonary Dysplasia - The five questions: Which cells, when, in which dose, to which patients via which route. Paediatr Respir Rev.24:54-9.
- Gronbach J, Shahzad T, Radajewski S, Chao CM, Bellusci S, et al. (2018) The Potentials and Caveats of Mesenchymal Stromal Cell-Based Therapies in the Preterm Infant. Stem cells internat. 2018:9652897.
- Wu X, Xia Y, Zhou O, Song Y, Zhang X, et al. (2020) Allogeneic human umbilical cord-derived mesenchymal stem cells for severe bronchopulmonary dysplasia in children: study protocol for a randomized controlled trial (MSC-BPD trial). Trials. 21(1):125.
- Greenough A. (2012) Long term respiratory outcomes of very premature birth (<32 weeks). Semin Fetal Neonatal Med. 17(2):73-6.
- Bhandari A, Panitch H. (2018) An update on the post-NICU discharge management of bronchopulmonary dysplasia. Semin Perinatol. 42(7):471-77.
- Möbius MA, Freund D, Vadivel A, Koss S, McConaghy S, et al. (2019) Oxygen Disrupts Human Fetal Lung Mesenchymal Cells. Implications for Bronchopulmonary Dysplasia. Am J Respir Cell Mol Biol. 60(5):592-600.
- Wang Y, Long W, Cao Y, Li J, You L, et al. (2020) Mesenchymal stem cell-derived secretomes for therapeutic potential of premature infant diseases. Bioscience rep. 40(5):BSR20200241.
- Fung ME, Thébaud B. (2014) Stem cell-based therapy for neonatal lung disease: it is in the juice. Pediatric res. 75(1-1):2-7.
- Ahn SY, Chang YS, Park WS. (2015) Stem cell therapy for bronchopulmonary dysplasia: bench to bedside translation. J Korean Med Sci. 30(5):509-13.
- Willis GR, Mitsialis SA, Kourembanas S. (2018) "Good things come in small packages": application of exosome-based therapeutics in neonatal lung injury. Pediatric res. 83(1-2):298-307.
- Strueby L, Thébaud B. (2018) Novel therapeutics for bronchopulmonary dysplasia. Current opinion in pediat. 30(3):378-83.
- Stoll BJ, Hansen NI, Bell EF, Shankaran S, Laptook AR, et al. (2010). Neonatal outcomes of extremely preterm infants from the NICHD Neonatal Research Network. Pediatrics. 126(3):443-56.
- Namba F. (2019) Mesenchymal stem cells for the prevention of bronchopulmonary dysplasia. Pediatr Int. 61(10), 945-50.
- Keszler M, Sant'Anna G. (2015) Mechanical Ventilation and Bronchopulmonary Dysplasia. Clinics in perinatol. 42(4):781-96.
- Day CL, Ryan RM. (2017) Bronchopulmonary dysplasia: new becomes old again! Pediatric res. 81(1-2):210-13.
- Sahni M, Bhandari V. (2020) Recent advances in understanding and management of bronchopulmonary dysplasia. 9:F1000 Faculty Rev-703.
- Mandell EW, Kratimenos P, Abman SH, Steinhorn RH. (2019) Drugs for the Prevention and Treatment of Bronchopulmonary Dysplasia. Clinics in perinatal. 46(2):291-310.
- Higgins RD, Jobe AH, Koso-Thomas M, Bancalari E, Viscardi RM, et al . (2018). Bronchopulmonary Dysplasia: Executive Summary of a Workshop. J. Pediatr. 197:300-08.
- Niver D. (2014) Bronchopulmonary dysplasia: structural challenges and stem cell treatment potential. Adv. Neonatal. Care ADV NEONAT CARE. 14(1):E1-E11.
- Ahn SY, Chang YS, Kim JH, Sung SI, Park WS. (2017) Two-Year Follow-Up Outcomes of Premature Infants Enrolled in the Phase I Trial of Mesenchymal Stem Cells Transplantation for Bronchopulmonary Dysplasia. J pediat. 185:49-54.e2.
- Ee MT, Thébaud, B. (2018) The Therapeutic Potential of Stem Cells for Bronchopulmonary Dysplasia: "It's About Time" or "Not so Fast". Current pediatric reviews. 14(4):227-38.
- Bancalari E, Jain D. (2019) Bronchopulmonary dysplasia: 50 years after the original description. Neonatol. 115(4):384-91.
- da Fonseca EB, Damião R, Moreira DA. Preterm birth prevention. Best Pract Res Clin Obstet Gynaecol. 69:40-9.
- Möbius MA, Thébaud B. Cell therapy for bronchopulmonary dysplasia: promises and perils. Paediatr Respir Rev. 20:33-41.
- Strueby L, Thebaud B. (2016) Mesenchymal Stromal Cell-Based Therapies for Chronic Lung Disease of Prematurity. Am J Perinatol. 33(11):1043-49.
- Behnke J, Kremer S, Shahzad T, Chao CM, Böttcher-Friebertshäuser E, et al. (2020). MSC Based Therapies-New Perspectives for the Injured Lung. J Clin Med. 9(3):682.
- Hussain M, Xu C, Lu M, Wu X, Tang L, et al. (2017). Wnt/β-catenin signaling links embryonic lung development and asthmatic airway remodeling. Biochimica et Biophysica Acta (BBA). Mol Basis Dis. 1863(12):3226-42.
- Pierro M, Thébaud B, Soll R. (2017) Mesenchymal stem cells for the prevention and treatment of bronchopulmonary dysplasia in preterm infants. Cochrane Database Syst Rev. 11(11):CD011932.
- Kang, M, Thébaud B. (2018) Stem cell biology and regenerative medicine for neonatal lung diseases. Pediat Res. 83(1-2):291-97.
- Sung DK, Chang YS, Ahn SY, Sung SI, Yoo HS, et al. (2015) Optimal Route for Human Umbilical Cord Blood-Derived Mesenchymal Stem Cell Transplantation to Protect Against Neonatal Hyperoxic Lung Injury: Gene Expression Profiles and Histopathology. PloS one, 10(8):e0135574.
- Sung DK, Chang YS, Ahn SY, Sung SI, Yoo HS, et al. (2015) Optimal Route for Human Umbilical Cord Blood-Derived Mesenchymal Stem Cell Transplantation to Protect Against Neonatal Hyperoxic Lung Injury: Gene Expression Profiles and Histopathology. PloS one. 10(8):e0135574.
- Collins A. (2020). Stem-cell therapy for bronchopulmonary dysplasia. Curr Opin Pediat. 32(2):210-5.
- Gheorghe CP, Bhandari V. (2015) Stem Cell Therapy in Neonatal Diseases. Indian J Pediatr. 82(7): 637-41.
- Pawelec K, Gładysz D, Demkow U, Boruczkowski D. (2015) Stem cell experiments moves into clinic: new hope for children with bronchopulmonary dysplasia. Inflammatory Disorders. 2014:47-53.
- Rüdiger M, Kirpalani H, Steinhorn R, Davis JM, Thebaud B. (2020). How to introduce MSC-based therapy for the developing lung safely into clinical care?. Pediat Res. 88(3):365-8..
- Ahn SY, Chang YS, Sung DK, Yoo HS, Sung SI, et al. (2015). Cell type-dependent variation in paracrine potency determines therapeutic efficacy against neonatal hyperoxic lung injury. Cytother. 17(8):1025-35.
- Northway Jr WH, Moss RB, Carlisle KB, Parker BR, Popp RL, et al. (1990) Late pulmonary sequelae of bronchopulmonary dysplasia. NEJM. 323(26):1793-99.
- Robin B, Kim YJ, Huth J, Klocksieben J, Torres M, et al. (2004) Pulmonary function in bronchopulmonary dysplasia. Pediat Pulmonol. 37(3):236-42.
- Jobe AH. (2011) The new bronchopulmonary dysplasia. Curr Opin Pediatr. 23(2):167-72.
- Fakhoury KF, Sellers C, Smith EB, Rama JA, Fan LL. (2010) Serial measurements of lung function in a cohort of young children with bronchopulmonary dysplasia. Pediat. 125(6):e1441-7.
- Aukland SM, Rosendahl K, Owens CM, Fosse KR, Eide GE, et al. (2009) Neonatal bronchopulmonary dysplasia predicts abnormal pulmonary HRCT scans in long-term survivors of extreme preterm birth. Thorax. 64(5):405-10.
- Karila C, Saulnier JP, Elie C, Taupin P, Scheinmann P, Le Bourgeois M, Waernessycle S, De Blic J. Alveolar hypoventilation during exercise in children with bronchopulmonary dysplasia. Rev Resp Dis. 25(3):303-12.
- Wong PM, Lees AN, Louw J, Lee FY, French N, et al. (2008) Emphysema in young adult survivors of moderate-to-severe bronchopulmonary dysplasia. Europ Resp J. 32(2):321-8.
- Thébaud B. (2018) Mesenchymal Stromal Cell Therapy for Respiratory Complications of Extreme Prematurity. Am J Perinatol. 35(06):566-9.
- Thébaud B. (2018) Stem cell-based therapies in neonatology: a new hope Arch Dis Child. 103(6):F583-8.
- Chen CM, Chou HC, Lin W, Tseng C. (2017) Surfactant effects on the viability and function of human mesenchymal stem cells: in vitro and in vivo assessment. Stem Cell Res Ther. 8(1):180.
- Chang YS, Ahn SY, Yoo HS, Sung SI, Choi SJ, et al. (2014) Mesenchymal stem cells for bronchopulmonary dysplasia: phase 1 dose-escalation clinical trial. J Pediatr. 164(5):966-72.
- Song N, Scholtemeijer M, Shah K. (2020) Mesenchymal stem cell immunomodulation: mechanisms ant therapeutic potential. Trends Pharmacol Sci. 41(9):653-64.
- Álvarez-Fuente M, Arruza L, Lopez-Ortego P, Moreno L, Ramírez-Orellana M, et al. (2018) Off-label mesenchymal stromal cell treatment in two infants with severe bronchopulmonary dysplasia: clinical course and biomarkers profile. Cytother. 20(11):1337-44.
- Lesage F, Jimenez J, Toelen J, Deprest J. (2018) Preclinical evaluation of cell-based strategies to prevent or treat bronchopulmonary dysplasia in animal models: a systematic review. J Matern Fetal Neonatal Med. 31(7):958-66.
- Augustine S, Avey MT, Harrison B, Locke T, Ghannad M, et al. (2017) Mesenchymal Stromal Cell Therapy in Bronchopulmonary Dysplasia: Systematic Review and Meta-Analysis of Preclinical Studies. Stem Cells Transl Med. 6(12):2079-93.
- Aslam M, Baveja R, Liang OD, Fernandez-Gonzalez A, Lee C, et al. (2009). Bone marrow stromal cells attenuate lung injury in a murine model of neonatal chronic lung disease. Am J Resp Crit Care. 180(11):1122:30.
- Zhang X, Wang H, Shi Y, Peng W, Zhang S, et al. (2012) Role of bone marrow-derived mesenchymal stem cells in the prevention of hyperoxia-induced lung injury in newborn mice. Cell Biol Internat. 36(6):589-94.
- Hansmann G, Fernandez-Gonzalez A, Aslam M, Vitali SH, Martin T, et al. (2012) Mesenchymal stem cell-mediated reversal of bronchopulmonary dysplasia and associated pulmonary hypertension. Pulm Circ. 2(2):170-81.
- Sutsko RP, Young KC, Ribeiro A, Torres E, Rodriguez M, et al. (2013) Long-term reparative effects of mesenchymal stem cell therapy following neonatal hyperoxia-induced lung injury. Pediat Res. 73(1):46-53.
- Pierro M, Ionescu L, Montemurro T, Vadivel A, Weissmann G, et al. (2013) Short-term, long-term and paracrine effect of human umbilical cord-derived stem cells in lung injury prevention and repair in experimental bronchopulmonary dysplasia. Thorax. 68(5):475-84.
- Ahn SY, Chang YS, Kim SY, Sung DK, Kim ES, et al. (2013) Long-term (postnatal day 70) outcome and safety of intratracheal transplantation of human umbilical cord blood-derived mesenchymal stem cells in neonatal hyperoxic lung injury. Yonsei Med J. 54(2):416-24.
- Wecht S, Rojas M (2016) Mesenchymal stem cells in the treatment of chronic lung disease. Respirology (Carlton, Vic.) 21(8):1366-75.
- Lim R, Malhotra A, Tan J, Chan ST, Lau S, et al. (2018) First-In-Human Administration of Allogeneic Amnion Cells in Premature Infants With Bronchopulmonary Dysplasia: A Safety Study. Stem Cells Transl Med. 7(9):628-35.
- Thébaud B, Kourembanas S. (2017) Can we cure bronchopulmonary dysplasia?. J Pediatr. 191:12-4.