Metabolic Syndrome Prevention Protocol: Musculoskeletal and Peripheral Nervous System Modalities
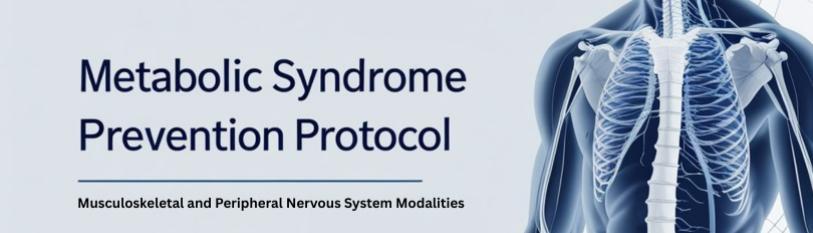
Mike KS Chan1, Stephanie Ann Sardar1, Mohd Shahril Abdul Rahim1, Michelle B. W. Wang1, Yuriy Nalapko1*, Simon Yefimov1, Jonathan Lakey2 and Thomas Skutella3
1European Wellness Biomedical Group, Germany
2Department of Surgery, University of California Irvine, Irvine, California, USA
3Institute for Anatomy and Cell Biology, Medical Faculty, University of Heidelberg, Heidelberg, Germany
*Corresponding author: Yuriy Nalapko, European Wellness Biomedical Group, Klosterstrasse 205, Edenkoben, Germany.
Citation: Chan MKS, Sardar SN, Rahim MSA, Wang MBW and Nalapko Y et al. Neuromuscular and Muscular-Skeletal Modulations as The Antiaging Strategy of Physical Adaptation in Non-Communicable Diseases. J Neurol Sci Res. 5(1):1-26.
Received: April 01, 2024 | Published: April 20, 2025
Copyright©️ 2025 genesis pub by Chan MKS, et al. CC BY-NC-ND 4.0 DEED. This is an open-access article distributed under the terms of the Creative Commons Attribution-Non-Commercial-No Derivatives 4.0 International License. This allows others distribute, remix, tweak, and build upon the work, even commercially, as long as they credit the authors for the original creation.
DOI:http://doi.org/10.52793/JNSR.2025.5(1)-44
Abstract
Introduction
Metabolic syndrome and type 2 diabetes represent rapidly escalating global health challenges, affecting over 830 million individuals worldwide. Conventional medicine approaches demonstrate limited efficacy in managing the complex pathophysiological mechanisms underlying glucose regulation disturbances. Peripheral nervous system and musculoskeletal activation exercises present promising bio-regenerative modalities for enhancing glycemic control through non-pharmacological pathways. This study investigates the acute effects of structured physical interventions on postprandial glucose metabolism, cardiovascular parameters, and physical adaptation processes.
Materials and Methods
The 49 participants of the investigation (28 male and 21 female) were exhibiting postprandial hyperglycemia (≥7.8 mmol/L) two hours after the last meal. Four seated exercises utilizing concentric, eccentric, and isometric contractions were implemented under a standardized 12-minute protocol at modified RPE 2-4 intensity. Capillary blood glucose measurements, systolic/diastolic blood pressure, and heart rate were systematically assessed at baseline and during five following phases spanning 15 minutes post-intervention.
Results
Male participants demonstrated significant reductions in postprandial glucose (20.4% decrease, p<0.001) from Baseline of 11.4 mmol/L to 9.1 mmol/L post-intervention. Female participants showed similar improvements (16.4% decrease, p<0.001) from 12.2 mmol/L to 10.2 mmol/L. The cardiovascular adaptations included statistically significant systolic blood pressure reductions in males (8 mmHg, p<0.001) and females (5 mmHg, p=0.030), with minimal heart rate variations. The glucose-lowering effects corresponded with the activation of GLUT4 translocation and AMPK-mediated insulin-independent pathways.
Conclusions
This research defines peripheral nervous system and musculoskeletal activation exercises as effective methods for acute glycemic regulation through specific physiological mechanisms. The significant reductions in postprandial hyperglycemia and blood pressure support the integration of these bio-regenerative approaches within holistic metabolic syndrome management protocols. Easily accessible, low-intensity interventions demonstrate strong value for individuals with mobility limitations or exercise intolerance. Further investigations examining synergistic effects with cell-based therapies and mitochondrial peptides promise optimized anti-aging outcomes and cardiometabolic health.
Keywords
Bio-regenerative medicine; Metabolic syndrome; Peripheral nervous system activation; Glucose metabolism; Glycemic control; Musculoskeletal modalities.
Introduction
Metabolic syndrome is a rapidly escalating global health concern. Diabetes is one of the leading non-communicable diseases (NCDs), affecting over 830 million people as of 2022. This number is projected to exceed 850 million by 2050. The increase is particularly significant in low- and middle-income countries, where access to early diagnosis and effective treatment remains limited [1]. Diabetes poses a high risk of severe complications such as cardiovascular disease, kidney failure, nerve damage, and vision loss. These complications not only reduce life expectancy but also accelerate the aging process by impairing the function of vital organs. Older adults are especially vulnerable, and with aging populations growing worldwide, healthcare systems face increasing strain [2]. In response to this challenge, the World Health Organization launched the Global Diabetes Compact in 2021, which focuses on prevention, early detection, and ensuring equitable access to care [3].
One of the strategies for the alternative prevention and management of metabolic syndrome and diabetes is the integ, ration of exercise or physical activity, which is personalized and adapted according to an individual's health status and physical capacity. Consistent and appropriately guided exercise or physical activity, such as walking, resistance exercises, and aerobics, can significantly improve glycemic control, increase insulin sensitivity, and support both heart and brain health [4,5]. These forms of exercise also help mitigate the physical decline often associated with diabetes and aging. When adapted to suit personal needs, these activities empower individuals to feel more energetic, capable, and in control of their well-being [6]. Nevertheless, many face challenges that limit their participation in regular physical activity. These include fatigue, complications related to diabetes, and broader socioeconomic issues such as limited access to safe environments, lack of time due to work or family commitments, and financial constraints [7]. Addressing these barriers through targeted education and supportive interventions is crucial [8]. By placing individualized physical activity at the heart of diabetes care, we not only improve disease management but also introduce a powerful anti-aging strategy that enhances both longevity and quality of life [9].
Peripheral nervous system and musculoskeletal activation exercises and glycemic control
Peripheral nervous system and musculoskeletal activation exercises, particularly those involving muscle contractions, have been shown to significantly improve glycemic control. These exercises enhance glucose uptake in skeletal muscle, even in the absence of insulin, and improve insulin sensitivity. This process plays an important role in managing blood glucose levels, especially for individuals dealing with insulin resistance or diabetes [10,11].
Skeletal muscle is the primary tissue responsible for insulin-stimulated glucose uptake, making it central to whole-body glucose metabolism and the regulation of blood glucose levels [12,13]. Under normal circumstances, insulin is secreted by the pancreas in response to elevated blood glucose levels. Insulin then binds to insulin receptors on muscle cells, signaling a cascade of events that facilitate glucose uptake. Once inside the muscle cells, glucose is either used immediately for energy during exercise or physical activity or stored as glycogen for later use. In healthy individuals, this process is essential for maintaining normal blood glucose levels [13].
However, even in the absence of insulin, skeletal muscle retains the ability to absorb glucose. This is where exercise or physical activity that contracts the muscle plays a pivotal role. By enhancing glucose uptake through muscle contractions, exercise compensates for impaired insulin signaling in individuals with insulin resistance or diabetes, helping to regulate blood glucose levels without depending on insulin function 100%. If insulin resistance weakens, muscle contraction acts as a supporting mechanism to regulate glucose [14].
Voluntary exercise (such as walking, cycling, or resistance training) can increase glucose uptake in skeletal muscle. During exercise, the contraction of muscle fibers facilitates the movement of glucose from the bloodstream into muscle cells. This process can occur independently of insulin, which is particularly beneficial for individuals with insulin resistance, where the typical insulin-mediated glucose uptake is impaired or weak. When skeletal muscles contract, they increase their energy demand, which in turn stimulates glucose uptake from the bloodstream. This process is primarily driven by the increased demand for energy during exercise. In voluntary exercise such as aerobic exercise or resistance training, muscle contractions directly induce glucose uptake into muscle cells, regardless of insulin availability. By promoting glucose uptake independently of insulin, exercise plays an important role in improving glycemic control, especially in individuals with insulin resistance or diabetes [15].
Additionally, muscle contractions themselves automatically activate a series of cellular functions that further enhance glucose uptake and energy production within muscle cells. Muscle contraction acts as a signal that triggers several intracellular pathways that increase the muscle's ability to absorb glucose and generate energy. These cellular processes are highly effective during exercise and provide immediate benefits for glucose regulation [16].
Mitochondrial dysfunction in metabolic syndrome
Metabolic syndrome, a multifaceted disorder characterized by abdominal obesity, insulin resistance, hypertension, and dyslipidemia, has emerged as a global health crisis, significantly escalating the risk of cardiovascular disease, type 2 diabetes, and non-alcoholic fatty liver disease [17]. The convergence of these metabolic abnormalities poses a substantial threat to public health, necessitating a comprehensive understanding of the underlying mechanisms driving this syndrome [18]. The interplay of genetic predispositions, environmental factors, and lifestyle choices contributes to the pathogenesis of metabolic syndrome, making it a complex and challenging condition to manage [19].
Mitochondrial dysfunction, characterized by impaired oxidative phosphorylation, reduced ATP production, and increased reactive oxygen species generation, has emerged as a central player in the development and progression of metabolic syndrome [20]. The recognition of mitochondrial function's crucial role in health and disease has grown significantly in recent decades, particularly in prevalent conditions like type 2 diabetes, cardiovascular disease, metabolic syndrome, cancer, and Alzheimer's disease [21]. Mitochondria are responsible for generating the majority of cellular energy through oxidative phosphorylation. Beyond energy production, mitochondria participate in a wide array of cellular processes, including calcium homeostasis, apoptosis, and the synthesis of essential biomolecules. It has become increasingly clear that abnormalities in mitochondrial structure and function are closely intertwined with the pathogenesis of metabolic syndrome [22].
Mitochondrial dysfunction in skeletal muscle, a major site of insulin-stimulated glucose disposal, contributes significantly to insulin resistance, a hallmark of metabolic syndrome. Defects in mitochondrial oxidative capacity impair the ability of skeletal muscle to efficiently oxidize fatty acids and glucose, leading to the accumulation of intramyocellular lipids and the activation of inflammatory signaling pathways, which interfere with insulin signaling [23]. This interplay between mitochondrial dysfunction and insulin resistance creates a vicious cycle, exacerbating the metabolic derangements associated with metabolic syndrome. The disruption of mitochondrial membrane potential can further exacerbate oxidative stress by inducing superoxide production within the electron transport chain, thereby contributing to cellular damage and vascular dysfunction [24]. Elevated levels of free fatty acids can impair insulin action in skeletal muscle and liver, leading to increased blood glucose concentration [25].
In the heart, mitochondrial dysfunction contributes to the development of diabetic cardiomyopathy, a condition characterized by impaired cardiac contractility and increased susceptibility to heart failure [26]. The compromised mitochondrial function in cardiomyocytes leads to reduced ATP production and increased reactive oxygen species generation, resulting in oxidative stress, inflammation, and ultimately, contractile dysfunction. Patients with insulin resistance exhibit a lower tolerance for ischemia and poor mechanical function, making them more susceptible to cardiovascular complications [27].
Metabolic syndrome and peripheral nerve dysfunction
The tight relationship between metabolic syndrome and the peripheral nervous system has garnered increasing attention in recent years, with emerging evidence suggesting that metabolic syndrome can exert detrimental effects on peripheral nerve structure and function. Peripheral neuropathy, a common complication of metabolic syndrome, is characterized by damage to peripheral nerves, leading to a diverse array of sensory, motor, and autonomic impairments that can significantly diminish quality of life. The pathogenesis of metabolic syndrome-associated peripheral neuropathy is multifactorial, involving a complex interplay of metabolic, vascular, and inflammatory mechanisms [22]. Dyslipidemia, a hallmark feature of metabolic syndrome, contributes to peripheral nerve damage by promoting atherosclerosis and microvascular dysfunction, thereby impairing nerve blood supply and oxygenation [28]. Elevated levels of triglycerides and low-density lipoprotein cholesterol can also directly damage nerve fibers, leading to demyelination and axonal degeneration. The interplay between metabolic syndrome and peripheral nerve health necessitates a comprehensive understanding of the underlying mechanisms to facilitate the development of targeted therapeutic interventions aimed at mitigating the neurological sequelae of this increasingly prevalent condition [29].
Insulin resistance plays a pivotal role in the pathogenesis of peripheral neuropathy through several interconnected mechanisms. Chronic hyperglycemia, resulting from insulin resistance, leads to the accumulation of advanced glycation end products in peripheral nerve tissues, which can directly damage nerve fibers and impair their function. Furthermore, insulin resistance can disrupt neuronal insulin signaling, leading to impaired nerve growth factor signaling and reduced nerve regeneration capacity [25]. Oxidative stress, characterized by an imbalance between the production of reactive oxygen species and antioxidant defense mechanisms, is a prominent feature of metabolic syndrome and plays a critical role in the development of peripheral neuropathy [30]. Elevated levels of oxidative stress can damage cellular components, including lipids, proteins, and DNA, leading to neuronal dysfunction and apoptosis. Moreover, oxidative stress can activate inflammatory pathways, further exacerbating nerve damage.
Mitochondrial peptides as a potential therapeutic approach for metabolic syndrome
The exploration of mitochondria-targeted therapeutics has gained momentum, offering potential avenues for manipulating mitochondrial function to achieve therapeutic benefits across various disease states. Within the network of mitochondrial components, a novel class of bioactive molecules, termed mitochondrial-derived peptides, has emerged as key players in cellular communication and metabolic regulation [31]. These peptides, encoded within the mitochondrial genome, exert a diverse array of biological activities, influencing cellular metabolism, stress responses, and aging processes. Given their involvement in critical cellular pathways, mitochondrial peptides represent promising therapeutic targets for combating metabolic syndrome, a cluster of metabolic abnormalities characterized by insulin resistance, obesity, hypertension, and dyslipidemia.
Targeting mitochondria emerges as a promising therapeutic strategy, particularly in the context of metabolic syndrome where mitochondrial dysfunction is a key feature. Current treatments for metabolic syndrome often address individual symptoms rather than targeting the underlying mitochondrial dysfunction [32]. Mitochondrial peptides offer a unique approach by directly modulating mitochondrial function and cellular metabolism, potentially addressing the root causes of the syndrome [17]. Elucidating the precise mechanisms by which mitochondrial peptides exert their effects is crucial for developing targeted therapies with minimal side effects.
European Wellness Biomedical Group employs bioregenerative technologies to prevent and treat non-communicable diseases like arterial hypertension. A primary approach involves lifestyle adjustments for all patients, incorporating physical activity, cell membrane rejuvenation through phospholipid therapy, mitochondrial replacement using organelle peptides, nano-sized organ-specific peptides, and anti-inflammatory plant-based bioactive molecules as key components of their anti-aging program. For repairing muscle tissue and the cardiovascular system, their clinics utilize natural bio-ingredients such as peptides, cell extracts, and tissue/organ-specific ingredients produced with specific pathogen-free technologies, recognized globally as a gold standard for safety in bioregenerative medicine.
Role of organ-specific peptides in prevention of metabolic syndrome
Peptides, short chains of amino acids, function as signaling molecules in the nervous system, influencing various physiological processes, including glucose metabolism, appetite regulation, and energy expenditure. Autonomic nervous system dysfunctions, especially increased sympathetic nervous system activity, have been shown to play a role in the development of metabolic syndrome and its cardiovascular complications [33]. Targeting these peptides in peripheral nerves could offer a novel approach to managing metabolic syndrome and mitigating its associated health risks.
Amylin and calcitonin gene-related peptide are members of the same peptide family that have garnered attention as potential therapeutic agents for metabolic diseases [34]. These peptides' capacity to augment insulin sensitivity, encourage weight loss, and complement incretin-based therapies positions them as appealing candidates for further research and clinical application [35].
The exploration of therapeutic interventions targeting peripheral nerve peptides are quite promising for addressing metabolic syndrome.
NOP – nanomized organ-specific peptides in prevention of metabolic syndrome
Amylin, also known as islet amyloid polypeptide, is a 37-amino acid peptide hormone co-secreted with insulin from pancreatic β-cells in response to nutrient stimuli, playing a crucial role in glucose homeostasis [36]. It exerts significant physiological effects on metabolic control, primarily acting as a regulator of meal-ending satiation, limiting the rate of gastric emptying, and reducing postprandial glucagon secretion. Amylin's primary mechanism in controlling food intake involves promoting meal-ending satiation, thereby reducing the size and duration of meals in a dose-dependent manner [37]. This effect is mediated through receptors in the area postrema of the brainstem, which is involved in the regulation of appetite and food intake.
Amylin's influence extends beyond appetite regulation, impacting gastric emptying by slowing the rate at which food is delivered from the stomach to the small intestine, leading to a blunting of postprandial glucose excursions. This action reduces the burden on pancreatic β-cells and contributes to improved glycemic control [36]. In addition, amylin inhibits postprandial glucagon secretion, further modulating glucose fluxes and preventing excessive hepatic glucose production following meals. The discovery of alternative, extra-pancreatic sources of amylin production raise intriguing possibilities regarding its broader physiological roles, especially in the context of food intake regulation, where it may function as an adiposity signal [38].
Calcitonin, a 32-amino acid peptide hormone primarily secreted by the parafollicular cells of the thyroid gland, has long been recognized for its role in calcium homeostasis, primarily by opposing the effects of parathyroid hormone and decreasing serum calcium levels. However, recent studies have illuminated calcitonin's broader implications in metabolic regulation, including its potential effects on insulin sensitivity, energy expenditure, and appetite control. Calcitonin receptors, members of the G-protein-coupled receptor superfamily, are expressed in various tissues beyond bone and kidney, including the brain, pancreas, and adipose tissue, suggesting a wider range of physiological actions.
The structural similarities between amylin, calcitonin, calcitonin gene-related peptide, and adrenomedullin, all sharing an N-terminal ring structure and amidated C-termini, hint at potential cross-talk and overlapping biological activities. These hormones interact with a family of receptors composed of a calcitonin receptor subunit and receptor activity-modifying proteins, forming distinct receptor subtypes with varying affinities for each peptide [39]. This complex interplay suggests complex regulatory mechanisms involving these peptides in metabolic processes [40].
While pharmacological interventions targeting calcitonin signaling have been primarily focused on bone metabolism, emerging evidence suggests that calcitonin analogs and related peptides may hold promise in addressing metabolic disorders. Specifically, the potential of utilizing amylin-based therapies, such as pramlintide, highlights the importance of understanding amylin's structure-function relationships for improved drug design [41]. Exploring the therapeutic potential of dual or multi-agonists that combine the actions of amylin and calcitonin receptor signaling could offer novel strategies for improving insulin sensitivity, promoting weight loss, and addressing the multifaceted metabolic derangements associated with metabolic syndrome.
Mechanisms of exercise-induced glucose uptake
GLUT4 translocation
One of the key mechanisms through which exercise increases glucose uptake is the translocation of glucose transporter type 4 (GLUT4) to the muscle cell membrane. GLUT4 is a protein embedded within intracellular vesicles in muscle cells. Under normal conditions, it is not available on the cell surface to facilitate glucose entry. However, during exercise, muscle contractions automatically activate signaling pathways that cause GLUT4 to move to the muscle cell membrane. This increases the muscle's capacity to absorb glucose from the bloodstream during and after exercise [13,12].
The presence of GLUT4 on the cell surface allows glucose to enter muscle cells more efficiently, providing the energy required for muscle contractions. This process is accelerated during physical activity, ensuring that glucose is used effectively, even in the absence of insulin. GLUT4 translocation is one of the most critical components of exercise-induced glucose uptake [11].
Insulin-independent pathways
In addition to insulin-dependent mechanisms, exercise also activates insulin-independent pathways that enhance glucose uptake. One such pathway involves adenosine monophosphate-activated protein kinase (AMPK), a key energy sensor within cells. When muscle cells contract during exercise, ATP (the cell’s energy currency) is broken down to generate energy, increasing the AMP-to-ATP ratio. This signals a low energy state, which activates AMPK. Once activated, AMPK triggers several cellular processes to restore energy balance, one of which is the promotion of GLUT4 translocation to the cell surface. This allows for greater glucose uptake and utilization, even without insulin [15]. Moreover, during muscle contraction, muscle cells actively pull glucose from the bloodstream as part of the energy system process. This is a crucial step, as muscle cells rely on glucose as a primary source of energy during exercise. The increased glucose uptake provides the necessary fuel for muscle contractions, ensuring that the muscle cells can sustain exercise. This process is particularly important during continuous exercise or physical activity, where glucose is rapidly utilized for energy [16].
AMPK also stimulates other metabolic pathways, such as increased fat oxidation, which further supports energy production during exercise. These insulin-independent mechanisms are particularly valuable in individuals with insulin resistance or diabetes disease, as they help enhance glucose uptake even when insulin signaling is impaired or weak [13].
Increased insulin sensitivity
Regular engagement in exercise training not only boosts glucose uptake during physical activity but also improves overall insulin sensitivity. Insulin sensitivity refers to how effectively the body responds to insulin. In individuals with insulin resistance, the muscle cells' ability to respond to insulin becomes diminished, leading to higher blood glucose levels. However, regular exercise training improves insulin sensitivity by enhancing the efficiency of insulin receptors and signaling pathways in muscle cells. Exercise induces several adaptations that increase insulin sensitivity, including an increase in the number of insulin receptors on muscle cells and improved intracellular signaling for glucose uptake. Additionally, regular physical activity improves the structural integrity of muscle tissue, such as increasing mitochondrial density, which enhances the cell's ability to utilize glucose and produce energy. As a result, the muscle cells become more responsive to insulin, requiring less insulin to transport glucose effectively [10,11]. Over time, this improved insulin sensitivity leads to better glucose control, reducing the need for insulin and lowering the risk of hyperglycemia. For individuals with insulin resistance, exercise can reverse or alleviate the condition, making it a cornerstone in the prevention and management of diabetes [11].
Neuromuscular and musculoskeletal activation exercises are effective in improving glycemic control through multiple mechanisms. By increasing glucose uptake into skeletal muscle via insulin-independent pathways, such as AMPK activation, and promoting the translocation of GLUT4 to the muscle cell membrane, exercise ensures that glucose is efficiently utilized.
Additionally, muscle contractions automatically trigger cellular processes within the muscle cells that actively pull glucose from the bloodstream as part of the energy system process. This allows muscle cells to meet their energy demands during exercise, ensuring that glucose is used effectively. Regular exercise not only enhances glucose uptake during physical activity but also improves overall insulin sensitivity, contributing to better long-term glucose regulation.
The combined effect of improved glucose uptake, insulin sensitivity, and overall metabolic function makes exercise a powerful tool in managing and preventing metabolic disorders such as diabetes disease. It provides an effective, non-pharmacological approach to improving blood glucose control and maintaining overall health [17].
Exercise intensity and its impact on glycemic control (glucose)
Post-meal exercise plays a crucial role in regulating blood glucose levels, offering significant benefits for individuals aiming to control their blood sugar, especially after meals (Chacko, 2016). Engaging in regular exercise, whether moderate or high-intensity, has been shown to reduce postprandial glucose spikes and improve overall metabolic health [18]. Research indicates that moderate-intensity exercise (modified RPE 2-4) can reduce blood glucose by an average of 2.0 mmol/L, while more vigorous activities (modified RPE 5-7) can lower glucose by an average of 3.0 mmol/L or more. By consistently incorporating these exercise types into a daily routine, individuals can enhance insulin sensitivity and experience long-term improvements in glycemic control [44].
Modified RPE and exercise intensity
The modified rating of perceived exertion (RPE) scale is an essential tool for determining exercise intensity based on how the body feels during physical activity [45]. It helps ensure that individuals select an intensity appropriate to their fitness level, promoting effective exercise without overexertion.
- Modified RPE 2-4 (light to moderate intensity): At this level, you will experience light perspiration, stable breathing, and the ability to talk comfortably while exercising. This intensity is ideal for people looking to improve glucose control without causing excessive strain on the body [46].
- Modified RPE 5-7 (moderate to vigorous intensity): As exercise intensity increases, breathing becomes heavier, sweat levels rise, and it becomes harder to hold a conversation. This level is effective for more significant glucose reduction and improving insulin sensitivity but requires careful attention to individual capabilities [47].
Understanding individual capabilities and preventing overexertion
It's essential to recognize and respect individual fitness levels when choosing exercise intensity. If the exercise intensity exceeds one’s capability, it can lead to overexertion, which occurs when the body is pushed beyond its ability to recover. Overexertion can have negative impacts, including increased inflammation, fatigue, and disrupted glucose regulation, potentially leading to fluctuations in blood glucose levels [49]. For individuals with diabetes or those prone to glucose instability, overexertion can trigger dangerous blood sugar responses:
- Hypoglycemia: Excessive or prolonged exercise without proper fuel or balance can lead to a sharp drop in blood glucose, resulting in symptoms such as dizziness, shaking, or confusion. This is especially risky for individuals managing diabetes [50].
- Hyperglycemia: On the other hand, very intense exercise without adequate preparation can temporarily raise blood glucose levels due to the body’s stress response, increasing the production of glucose by the liver [46]. To avoid these risks, it's essential to tailor exercise intensity to your personal fitness level. Overexertion hampers physical adaptation, the process through which the body adjusts to and benefits from exercise. When intensity is matched with individual capacity, physical adaptation occurs, leading to improvements in fitness, glucose control, and overall health, while minimizing the harmful effects of overtraining.
Selecting the right exercise intensity is key to maximizing the benefits of physical activity while preventing negative impacts on glucose levels. Regular, moderate-intensity exercise (modified RPE 2-4) is generally safe and effective for improving glycemic control, while higher intensities (modified RPE 5-7) can be beneficial when performed correctly [43,18]. However, it is critical to be mindful of your individual fitness level to prevent overexertion, which can lead to unstable blood glucose levels and hinder the body's ability to adapt to exercise. By understanding the right intensity for your body, you can optimize glycemic control, enhance metabolic health, and avoid the risks of hypoglycemia and hyperglycemia [46,44].
Peripheral nervous system and musculoskeletal activation exercises: Impact on cardiovascular health, metabolic health, and anti-aging
Peripheral nervous system and musculoskeletal activation exercises, when paired with effective glycemic control strategies, offer significant benefits for cardiovascular health, metabolic function, and anti-aging. These exercises enhance insulin sensitivity, promote glucose uptake, improve muscle function, and support physical adaptation to meet the demands of regular physical activity. These are key factors in disease prevention, healthier aging, and better overall physical performance [50,51,52].
Cardiovascular health
Neuromuscular and musculoskeletal activation exercises are instrumental in improving cardiovascular health by targeting risk factors such as insulin resistance, high blood pressure, and poor vascular function [50]. Activities like resistance training and aerobic exercises increase insulin sensitivity, allowing the body to process glucose more efficiently [51]. This ultimately reduces the risk of cardiovascular diseases such as heart attacks and strokes [52]. During exercise, muscle contractions help promote glucose uptake in skeletal muscles, lowering blood glucose levels and reducing insulin resistance [53]. Regular physical activity also enhances vascular health by improving blood vessel elasticity and reducing blood pressure through better endothelial function [54]. These improvements in cardiovascular function, along with enhanced physical adaptation, improve the body's ability to perform and sustain physical activity over time [55].
Metabolic health
When paired with glycemic control strategies such as a balanced diet, neuromuscular and musculoskeletal exercises significantly enhance metabolic health. These exercises help regulate blood glucose by increasing insulin sensitivity and glucose utilization in muscle tissue [56]. The activation of glucose transporters during exercise aids in the uptake of glucose into the muscles, further supporting glucose control and reducing the risk of developing insulin resistance, metabolic syndrome and diabetes disease [53]. Resistance training increases muscle mass, which plays a critical role in glucose disposal and optimizes glucose uptake when insulin is active [52]. Exercise also helps reduce visceral fat, a major contributor to metabolic syndrome, by increasing energy expenditure and promoting fat oxidation [56]. These adaptations not only improve body composition and metabolic efficiency but also enhance the body's physical adaptability, enabling individuals to better sustain and recover from physical activity while reducing fatigue and injury risk [57,58].
Anti-aging
Peripheral nervous system and musculoskeletal activation exercises are crucial for preventing sarcopenia, the loss of muscle mass and strength that accelerates with age. Regular resistance training stimulates muscle protein synthesis and promotes the growth and maintenance of muscle tissue [57]. This is vital for maintaining mobility, balance, and functional independence, particularly in older adults [58]. Muscle contractions during exercise activate important cellular signaling pathways such as the mTOR pathway, which plays a key role in protein synthesis and muscle repair [59]. Additionally, regular exercise improves muscle power and contraction velocity, both essential for performing everyday tasks like walking, climbing stairs, and maintaining balance [17]. These improvements represent key aspects of physical adaptation, helping the body remain capable, agile, and responsive to physical demands with age. This not only supports daily physical activity but also reduces the risk of falls and other injuries related to aging [51].
Peripheral nervous system and musculoskeletal activation exercises, when paired with effective glycemic control, offer a holistic approach to improving cardiovascular health, optimizing metabolic function, and promoting healthy aging. Through enhanced glucose uptake, improved insulin sensitivity, increased physical adaptation, and the prevention of muscle mass loss, these exercises contribute to long-term health and vitality [52,53]. Regular physical activity enhances quality of life and promotes longevity by supporting heart health, improving metabolic function, strengthening muscles, and enabling the body to adapt and respond more effectively to physical activity [59]. This makes exercise a cornerstone of healthy aging, disease prevention, and sustained functional capacity [57].
Materials and Methods
This study adopts a quantitative research design focused on evaluating the physiological effects of peripheral nervous system and musculoskeletal activation exercises on glycemic control—measured through capillary blood glucose—and cardiovascular indicators such as blood pressure and heart rate (Hopkins, 2000). A total of 49 participants, comprising 28 males and 21 females, were recruited based on specific inclusion criteria. Eligible participants had a postprandial blood glucose level of 7.8 mmol/L or higher, measured at least two hours after their last meal, during which only plant-based water was permitted. All participants had a documented history of prediabetes or diabetes [60]. To ensure data validity and participant safety, strict exclusion criteria were applied. These included individuals who were pregnant or breastfeeding, those with severe psychiatric disorders or advanced medical conditions such as cancer or organ failure, participants currently involved in conflicting clinical trials, and individuals with musculoskeletal or neurological impairments that could interfere with exercise execution or recovery [61]. These criteria were implemented to ensure participants were physically and mentally fit to safely engage in the prescribed exercise interventions, thus minimizing confounding variables and maintaining the integrity of the study outcomes [62,63,17].
Phase 1: Pre-data collection and procedure
Participants first received a detailed briefing on the study’s goals, procedures, risks, and eligibility, followed by informed consent. After a 10-minute seated rest to stabilize cardiovascular function, baseline blood pressure (BP) was measured using an automated cuff [64,65]. Immediately afterward, a capillary blood glucose test was performed via finger prick to capture resting glycemic levels before exercise [60,61].
Next, anthropometric measurements (e.g., height) were taken, and body composition was analyzed using bioelectrical impedance analysis (BIA) (Ward, 2018). Participants then completed a supervised practice session of the exercise routine to ensure safe execution and identify any physical limitations [66,67].
To minimize confounding variables, participants were instructed to avoid food or stimulant beverages (e.g., caffeine) for at least two hours before data collection, and only consume plant-based water post-meal [68]. Non-compliant individuals were excluded.
Exercise intensity was guided by the Modified Rating of Perceived Exertion (RPE) scale, targeting a level between 2 and 4 (light to moderate) [69,70,17]. Practitioners monitored participants' breathing, facial expressions, and movement quality to ensure they remained within this range [71]. Exercises were stopped if the participant’s RPE exceeded 4 or signs of overexertion appeared [72,73,17].
Lastly, the participants’ ability to complete all four exercises safely was evaluated. Any exercise that caused overexertion or reduced the participant’s range of motion by more than 60% was excluded for that individual [17,74].
Phase 2: Neuromuscular activation exercise
Participants engaged in a 12-minute routine consisting of four seated exercises designed to improve muscle strength, endurance, and recovery. Each exercise involved concentric, eccentric, and isometric contractions to activate both slow- and fast-twitch muscle fibers [17,75]. Participants completed 2 sets of 8 repetitions per exercise, with each rep including an 8-second isometric hold. Exercise intensity was kept within an RPE of 2–4 to prevent fatigue [76]. Rest between sets was 60–90 seconds, but there was no rest between exercises to maintain continuous muscle engagement and cardiovascular stimulation [77,78].
The Phase 2 intervention involved a structured protocol of four seated exercises designed to strengthen and stabilize specific muscle groups using concentric, eccentric, and isometric contractions. Each exercise was performed alternately with both limbs, included an 8-second isometric hold, and was completed in a seated posture to ensure safety and accessibility [79,80,81].
Routine 1: Seated alternate knee tuck & ankle flexion
Participants began with arms resting on shoulders for posture stabilization, then performed alternating knee lifts and ankle flexions, engaging hip flexors, abdominals, and core through controlled movements and static holds 8 seconds [82,80,84,81,85].
Routine 2: Seated chest fly & handgrip
Participants began with open hands at shoulder level, followed by handgrip squeezing (50–80% strength), outward arm movements, and a backward pull with 8-second hold, activating the forearm flexors, deltoids, pectorals, rhomboids, and latissimus dorsi for upper body stability and grip strength [86-91].
Routine 3: Seated alternate leg extension & ankle flexion
Required a starting posture with arms stabilizing the upper body, followed by alternating leg extensions with simultaneous ankle dorsiflexion hold for 8 seconds, engaging quadriceps, tibialis anterior, hip flexors, and core for lower limb strength.
Routine 4: Seated 180 front raise
Began with open hands, followed by a controlled handgrip squeeze and a 180-degree upward arm raise with elbows locked and arms parallel to ears and hold for 8 second. This activated the deltoids, trapezius, rhomboids, and core stabilizers to promote upper body posture and shoulder function [87,82,92].
Phase 3: Post-exercise recovery monitoring
Following the exercise session, participants remained seated while undergoing structured recovery monitoring. Blood pressure and heart rate were measured at five specific intervals: 3–4, 5–6, 7–8, 9–10, and 11–12 minutes post-exercise. These stages captured the physiological response to light-to-moderate intensity activity (RPE ≤ 4) [93,94,17]. Initially, cardiovascular markers remained elevated as the body began clearing metabolic waste (Phase 1) [93-95,17]. In the early stages of recovery (Phase 2), parasympathetic activity increased, leading to reduced BP and HR [96,94,95,17]. By mid-recovery (Phase 3), these values approached baseline, reflecting effective cardiovascular recovery [96,94,95,17]. In the late and near-full recovery phases (Phases 4 and 5), BP and HR continued to decline, stabilizing at pre-exercise levels [96,94,97,95,17]. This protocol helped track the gradual return to homeostasis and provided insights into cardiovascular efficiency post-activity [96,94,9617].
Phase 4: Post-exercise glucose measurement
At 13–15 minutes post-exercise, a capillary finger-prick blood glucose test was administered to evaluate participants’ glycemic response after neuromuscular activation. This timing allowed the body sufficient time to enter recovery while capturing the acute impact of exercise on glucose regulation, offering critical insight into post-exercise metabolic changes [98-102].
Results
The study utilized paired t-tests to analyze pre- and post-exercise differences in systolic and diastolic blood pressure, heart rate, and glucose levels. Changes were calculated using the percentage change formula: (Post − Pre) / Pre × 100, as outlined by ScienceDirect (2023). A negative percentage indicated a decrease in values post-intervention, and significance was determined at p < 0.05. This statistical approach helped evaluate the effectiveness of the intervention in improving cardiovascular and metabolic markers, assuming data normality [103].
Table 1: Male Descriptive Statistic: Anthropometry and Body Composition (N=28).
Age (yrs. old) | Standing height (cm) | Body weight (kg) | Fat free mass (kg) | Skeletal muscle mass (kg) | Percentage body fat (%) | |
Highest | 71 | 186 | 109.8 | 73.2 | 40.8 | 42.8 |
Average | 59 | 167 | 79.2 | 54.8 | 30.2 | 30.2 |
Lowest | 37 | 152 | 49.8 | 37.7 | 20.1 | 9.6 |
Standard deviation (SD) | 8.5 | 8.45 | 14.03 | 7.82 | 4.61 | 6.82 |
Table 2: Female Descriptive Statistic: Anthropometry and Body Composition (N=21).
Age (yrs. old) | Standing height (cm) | Body weight (kg) | Fat free mass (kg) | Skeletal muscle mass (kg) | Percentage body fat (%) | |
Highest | 73 | 167 | 111.5 | 59.1 | 32.7 | 47 |
Average | 55 | 156 | 67.8 | 41.7 | 22.4 | 37.7 |
Lowest | 28 | 150 | 48.6 | 35 | 18.4 | 25.8 |
Standard deviation (SD) | 12.95 | 4.44 | 14.25 | 6.14 | 3.65 | 6.12 |
Table 3: Male descriptive statistic: Blood pressure and glucose (N=28).
Time frame | Pre – Monitoring | Post – Monitoring | ||||||||||||||||||
Blood Pressure | Pre GLU | Blood Pressure | ||||||||||||||||||
3 - 4 min | Blood Pressure | |||||||||||||||||||
5 - 6 min | Blood Pressure | |||||||||||||||||||
7 - 8 min | Blood Pressure | |||||||||||||||||||
9 - 10 min | Blood Pressure | |||||||||||||||||||
11 - 12 min | Post GLU | |||||||||||||||||||
SYS | DIA | HR | SYS | DIA | HR | SYS | DIA | HR | SYS | DIA | HR | SYS | DIA | HR | SYS | DIA | HR | |||
Highest | 168 | 105 | 105 | 19.9 | 159 | 102 | 116 | 161 | 104 | 114 | 157 | 101 | 115 | 154 | 100 | 117 | 151 | 104 | 118 | 18.9 |
Average | 135 | 82 | 78 | 11.4 | 135 | 82 | 81 | 131 | 81 | 80 | 128 | 81 | 80 | 127 | 80 | 80 | 127 | 80 | 80 | 9.1 |
Lowest | 109 | 62 | 56 | 7.9 | 108 | 62 | 57 | 99 | 56 | 55 | 100 | 62 | 59 | 98 | 57 | 55 | 102 | 55 | 52 | 5.1 |
Standard deviation (SD) | 14.98 | 10.37 | 13.99 | 3.36 | 14.24 | 9.35 | 15.81 | 15.47 | 10.09 | 14.81 | 14.09 | 8.94 | 14.4 | 14.75 | 10.06 | 14.84 | 14.1 | 9.56 | 14.7 | 3.38 |
Remarks: SYS – systolic BP (mmHg), DIA – diastolic BP (mmHg), HR – heart rate (bpm), GLU – blood glucose (mmol/L).
The male participants (N=28), averaging 59 years old, 167 cm tall, 79.2 kg in weight, and 30.2% body fat, showed a decrease in systolic blood pressure from 135 to 127 mmHg, diastolic from 82 to 80 mmHg, a slight rise in heart rate from 78 to 80 bpm, and a drop in glucose levels from 11.4 to 9.1 mmol/L.
Table 4: Female descriptive statistic: Blood pressure and glucose (N=21).
Time frame | Pre – Monitoring | Post – Monitoring | ||||||||||||||||||
Blood Pressure | Pre GLU | Blood Pressure | ||||||||||||||||||
3 - 4 min | Blood Pressure | |||||||||||||||||||
5 - 6 min | Blood Pressure | |||||||||||||||||||
7 - 8 min | Blood Pressure | |||||||||||||||||||
9 - 10 min | Blood Pressure | |||||||||||||||||||
11 - 12 min | Post GLU | |||||||||||||||||||
SYS | DIA | HR | SYS | DIA | HR | SYS | DIA | HR | SYS | DIA | HR | SYS | DIA | HR | SYS | DIA | HR | |||
Highest | 176 | 101 | 115 | 19.8 | 175 | 105 | 115 | 173 | 99 | 112 | 166 | 97 | 114 | 167 | 94 | 113 | 161 | 88 | 114 | 17 |
Average | 128 | 78 | 81 | 12.2 | 129 | 79 | 82 | 127 | 77 | 81 | 124 | 77 | 81 | 123 | 75 | 81 | 122 | 74 | 80 | 10.2 |
Lowest | 102 | 64 | 66 | 7.8 | 105 | 65 | 71 | 105 | 65 | 69 | 104 | 64 | 71 | 101 | 59 | 70 | 102 | 58 | 69 | 4.9 |
Standard deviation (SD) | 20.43 | 9.29 | 10.9 | 3.75 | 19.26 | 9.99 | 11.11 | 18.28 | 8.35 | 11.03 | 17.31 | 8.81 | 10.51 | 16.97 | 8.3 | 9.56 | 16.45 | 6.98 | 10.27 | 3.45 |
Remarks: SYS – systolic BP (mmHg), DIA – diastolic BP (mmHg), HR – heart rate (bpm), GLU – blood glucose (mmol/L).
Female participants (N=21), averaging 55 years old, 156 cm tall, 67.8 kg, and 37.7% body fat, showed a drop in systolic pressure from 128 to 122 mmHg, diastolic from 78 to 74 mmHg, stable heart rate around 81 bpm, and reduced glucose levels from 12.2 to 10.2 mmol/L.
Table 5: Male paired t-tests (two-tail) result: Blood pressure and glucose (N=28).
SYS | t-test: Pair sample | |||||
mean / average | ||||||
Time Interval | Pre Average SYS (mmHg) | Post Average SYS (mmHg) | Average Difference: Post - Pre | % Average Difference: Post - Pre | t Stat SYS | P(T<=t) SYS (Two-tail) |
Pre BP | 135 | - | - | - | - | |
Post BP 3 - 4 min | 135 | 135 | -1 | 0 | 0.3 | 0.766 |
Post BP 5 - 6 min | 135 | 131 | -4 | -3 | 2.95 | 0.007 * |
Post BP 7 - 8 min | 135 | 128 | -7 | -5 | 5.1 | 0.000 * |
Post BP 9 - 10 min | 135 | 127 | -8 | -6 | 5.44 | 0.000 * |
Post BP 11 - 12 min | 135 | 127 | -8 | -6 | 5.64 | 0.000 * |
DIA | t-test: Pair sample mean | |||||
Time Interval | Pre Average DIA (mmHg) | Post Average DIA (mmHg) | Average Difference: Post - Pre | % Average Difference: Post - Pre | t Stat SYS | P(T<=t) SYS (Two-tail) |
Pre BP | 82 | - | - | - | - | - |
Post BP 3 - 4 min | 82 | 82 | -1 | -1 | 0.51 | 0.615 |
Post BP 5 - 6 min | 82 | 81 | -1 | -1 | 1.16 | 0.255 |
Post BP 7 - 8 min | 82 | 81 | -1 | -2 | 1.93 | 0.065 |
Post BP 9 - 10 min | 82 | 80 | -2 | -3 | 2.85 | 0.008 * |
Post BP 11 - 12 min | 82 | 80 | -3 | -3 | 2.95 | 0.006 * |
HR | t-test: Pair sample mean | |||||
Time Interval | Pre Average HR (mmHg) | Post Average HR (mmHg) | Average Difference: Post - Pre | % Average Difference: Post - Pre | t Stat HR | P(T<=t) HR (Two-tail) |
Pre BP | 78 | - | - | - | - | - |
Post BP 3 - 4 min | 78 | 81 | 3 | 4 | -3.49 | 0.002 * |
Post BP 5 - 6 min | 78 | 80 | 2 | 3 | -2.45 | 0.021 * |
Post BP 7 - 8 min | 78 | 80 | 2 | 3 | -2.56 | 0.016 * |
Post BP 9 - 10 min | 78 | 80 | 2 | 3 | -1.94 | 0.063 |
Post BP 11 - 12 min | 78 | 80 | 2 | 3 | -2.28 | 0.030 * |
GLU | t-test: Pair sample mean | |||||
Pre Average GLU (mmol/l) | Post Average GLU (mmol/l) 13 - 15 Min | Average Difference: Post - Pre | % Average Difference: Post - Pre | t Stat HR | P(T<=t) HR (Two-tail) | |
11.4 | 9.1 | -2.3 | -20.4 | 12.03 | 0 |
Remarks: Two-tailed test, significance level α = 0.05. Asterisks (*) indicate p < 0.05. SYS – systolic BP (mmHg), DIA – diastolic BP (mmHg), HR – heart rate (bpm), GLU – blood glucose (mmol/L).
The paired t-test results show that pre-monitoring blood pressure for males averaged 135 mmHg systolic and 82 mmHg diastolic, which is considered borderline hypertension stage 1. Systolic blood pressure showed a significant reduction starting at 5–6 minutes (p = 0.007) and continued through 7–8, 9–10, and 11–12 minutes (p < 0.001). The average systolic reduction was 8 mmHg (a 6% drop). For diastolic blood pressure, significant reductions began at 9–10 minutes (p = 0.008) and continued at 11–12 minutes (p = 0.006), with a total reduction of 3 mmHg (a 3.7% drop).
Regarding heart rate, the average pre-monitoring heart rate was 78 bpm. A slight increase in heart rate was observed, reaching 80 bpm at 3–4 minutes, with a consistent value of 80 bpm throughout subsequent intervals, indicating a modest 2 bpm increase (a 2.6% rise), which was statistically significant at multiple time points, particularly at 3–4 minutes (p = 0.002), 5–6 minutes (p = 0.021), and 7–8 minutes (p = 0.016).
Regarding glucose levels, the pre-monitoring average was 11.4 mmol/L, with a significant decrease of 2.3 mmol/L (a 20.4% drop) to 9.1 mmol/L (p < 0.001), indicating a notable reduction.
These findings suggest that systolic blood pressure starts to significantly drop after 5 minutes (p = 0.007), diastolic pressure reduces meaningfully after 9 minutes (p = 0.008), heart rate shows a slight but significant increase throughout (particularly at 3–4 minutes, p = 0.002), and glucose levels decrease significantly (p < 0.001) throughout the monitoring period.
Table 6: Female paired t-tests (two-tail) result: Blood pressure and glucose (N=21).
SYS | t-test: Pair sample | |||||
mean / average | ||||||
Time Interval | Pre Average SYS (mmHg) | Post Average SYS (mmHg) | Average Difference: Post - Pre | % Average Difference: Post - Pre | t Stat SYS | P(T<=t) SYS (Two-tail) |
Pre BP | 128 | - | - | - | - | |
Post BP 3 - 4 min | 128 | 129 | 2 | 1 | -0.7 | 0.494 |
Post BP 5 - 6 min | 128 | 127 | -1 | -1 | 0.41 | 0.683 |
Post BP 7 - 8 min | 128 | 124 | -4 | -3 | 1.73 | 0.098 |
Post BP 9 - 10 min | 128 | 123 | -4 | -3 | 1.93 | 0.067 |
Post BP 11 - 12 min | 128 | 122 | -5 | -4 | 2.34 | 0.030 * |
DIA | t-test: Pair sample mean | |||||
Time Interval | Pre Average DIA (mmHg) | Post Average DIA (mmHg) | Average Difference: Post - Pre | % Average Difference: Post - Pre | t Stat SYS | P(T<=t) SYS (Two-tail) |
Pre BP | 78 | - | - | - | - | - |
Post BP 3 - 4 min | 78 | 79 | 1 | 1 | -0.7 | 0.494 |
Post BP 5 - 6 min | 78 | 77 | -1 | -1 | 0.37 | 0.713 |
Post BP 7 - 8 min | 78 | 77 | -1 | -1 | 0.71 | 0.487 |
Post BP 9 - 10 min | 78 | 75 | -3 | -3 | 2.04 | 0.055 |
Post BP 11 - 12 min | 78 | 74 | -4 | -5 | 3.14 | 0.005 * |
HR | t-test: Pair sample mean | |||||
Time Interval | Pre Average HR (mmHg) | Post Average HR (mmHg) | Average Difference: Post - Pre | % Average Difference: Post - Pre | t Stat HR | P(T<=t) HR (Two-tail) |
Pre BP | 81 | - | - | - | - | - |
Post BP 3 - 4 min | 81 | 82 | 2 | 2 | -1.86 | 0.077 |
Post BP 5 - 6 min | 81 | 81 | 1 | 1 | -0.96 | 0.348 |
Post BP 7 - 8 min | 81 | 81 | 0 | 0 | -0.34 | 0.735 |
Post BP 9 - 10 min | 81 | 81 | 0 | 0 | -0.18 | 0.862 |
Post BP 11 - 12 min | 81 | 80 | 0 | 0 | 0.23 | 0.818 |
GLU | t-test: Pair sample mean | |||||
Pre Average GLU (mmol/l) | Post Average GLU (mmol/l) 13 - 15 Min | Average Difference: Post - Pre | % Average Difference: Post - Pre | t Stat HR | P(T<=t) HR (Two-tail) | |
12.2 | 10.2 | -2 | -16.4 | 7 | 0.000 * |
Remarks: Two-tailed test, significance level α = 0.05. Asterisks (*) indicate p < 0.05. SYS – systolic BP (mmHg), DIA – diastolic BP (mmHg), HR – heart rate (bpm), GLU – blood glucose (mmol/L).
The paired t-test results for females show that pre-monitoring blood pressure averaged 128 mmHg systolic and 78 mmHg diastolic, which is considered within normal range or elevated. Systolic blood pressure showed significant reduction started at 11–12 minutes (p = 0.030), with a total reduction of 5 mmHg (a 4% drop). For diastolic blood pressure, there were no significant changes initially, but a significant reduction began at 9–10 minutes (3 mmHg, p = 0.055) and continued at 11–12 minutes (4 mmHg, a 5% drop, p = 0.005).
Regarding heart rate, the pre-monitoring average was 81 bpm, with no significant change during the monitoring period. The heart rate remained stable at 81 bpm, indicating no meaningful variation.
Regarding glucose levels, the pre-monitoring average was 12.2 mmol/L, with a significant decrease of average 2.0 mmol/L (a 16.4% drop) to 10.2 mmol/L (p < 0.001), indicating a notable reduction.
In summary, systolic blood pressure showed a significant decrease only after 11 minutes (p = 0.030), diastolic blood pressure decreased meaningfully after 9 minutes (p = 0.055), heart rate remained stable, and glucose levels showed a consistent and significant reduction throughout the monitoring period (p < 0.001).
Discussion
This study evaluated the effects of peripheral nervous system and musculoskeletal activation exercises on glycemic control, cardiovascular responses, and potential anti-aging outcomes in adult male and female participants with elevated blood glucose and blood pressure levels. The findings demonstrated that short bouts of postprandial, low-to-moderate intensity muscle activation exercises can significantly reduce blood glucose, systolic and diastolic blood pressure, with minor or stable changes in heart rate. These outcomes support and extend current evidence on the role of targeted peripheral nervous system and musculoskeletal activation exercises in prevention of metabolic syndrome and promoting healthy aging.
The study found significant reductions in blood glucose levels for both male (20.4% drop) and female (16.4% drop) participants following brief neuromuscular and musculoskeletal activation exercises performed approximately two hours post-meal. This aligns strongly with existing research, which highlights that muscle contraction stimulates glucose uptake independently of insulin through mechanisms such as GLUT4 translocation and AMPK pathway activation [10,12,104].
The reduction observed in this study mirrors the glucose-lowering effects seen in moderate-intensity physical activity protocols, where postprandial glucose spikes can be reduced by 2–3 mmol/L [104,41]. The exercises in this study were intentionally designed to be of lower intensity, around a Rating of Perceived Exertion (RPE) of 2–4, which reflects a mild to moderate intensity. Research by Schubert-Olesen et al. (2022) and Mateus et al. (2025) suggests that physical activity of this intensity, even for short durations, can significantly improve glucose regulation. Schubert-Olesen et al. (2022) further discuss the role of continuous glucose monitoring during moderate-intensity activity, emphasizing its potential to track real-time changes in blood glucose levels, which can enhance understanding of how such exercises influence glycemic control.
These findings reinforce the growing consensus that non-pharmacological strategies like short, post-meal physical activity bouts can be critical in enhancing glycemic control, reducing reliance on insulin, and potentially reversing insulin resistance over time [17,16]. Importantly, these exercises help maintain glucose homeostasis through both immediate (contraction-driven glucose uptake) and long-term (improved insulin sensitivity) effects.
Significant reductions in systolic and diastolic blood pressure were observed, particularly in male participants—where systolic BP dropped from 135 to 127 mmHg (a 6% reduction, p < 0.001), and diastolic from 82 to 80 mmHg. For females, systolic pressure decreased from 128 to 122 mmHg (4% reduction, p = 0.030) and diastolic from 78 to 74 mmHg (5% drop, p = 0.005). These findings are consistent with prior studies that show structured exercise, even in brief forms, can reduce arterial stiffness and improve endothelial function, leading to lower blood pressure [51,52,54,76,74,62].
The more immediate and pronounced effect in males may be attributed to their higher baseline levels (borderline hypertensive range), where initial improvements are often more dramatic. This supports existing evidence indicating that individuals with elevated baseline systolic BP tend to experience greater benefits from moderate physical activity [58,17,95,105].
In contrast, the modest increase in heart rate in male participants (from 78 to 80 bpm, a 2.6% rise) and the stable heart rate in females suggest that these exercises operate at a low-to-moderate intensity—consistent with a modified RPE of 2–4. This is crucial for safety and sustainable implementation, as excessive exertion can paradoxically increase glucose levels or provoke cardiovascular strain [46,48,106,43,107,17]. The minor heart rate elevation further indicates that the activity was well within safe thresholds for this demographic, promoting cardiovascular adaptations without overloading the system [93,97,08].
The time-course analysis of the blood pressure response indicated that systolic reduction in males began significantly at 5–6 minutes, while diastolic pressure changed meaningfully after 9–10 minutes. For females, the systolic effect became significant after 11–12 minutes. This suggests a threshold duration required to elicit physiological adaptations such as improved vascular tone and nitric oxide-mediated vasodilation, which are time-dependent [50,94].
These findings provide practical insights for the structuring of brief, post-meal routines: 10–12 minutes of activation exercises may be an optimal window for maximizing glycemic and cardiovascular benefits while maintaining adherence and safety. This also supports the WHO and ADA’s recommendations of at least 150 minutes of moderate physical activity per week, distributed throughout the day to manage glucose and cardiovascular health [6,2].
The preservation and activation of skeletal muscle through brief, targeted exercise is essential in preventing sarcopenia, supporting mobility, and reducing falls in older adults [57-58]. The findings of this study show that simple exercises, even when performed in a chair, can stimulate muscle contraction and trigger beneficial adaptations, such as increased insulin sensitivity, mitochondrial biogenesis, and protein synthesis via pathways like AMPK and mTOR [50,17,53].
By promoting muscular function, vascular health, and glucose regulation simultaneously, these exercises serve as a multi-targeted intervention not only for diabetes management but also for delaying physical decline associated with aging. This supports a paradigm shift toward personalized, micro-dose exercise prescriptions that improve healthspan as much as lifespan [73,4].
A key strength of this study lies in the real-life applicability of the exercise protocol. Many individuals face barriers to regular physical activity due to socioeconomic limitations, lack of access to safe environments, time constraints, or existing health conditions [7,108]. This research addresses those challenges by offering a simple, equipment-free routine that can be performed at home in under fifteen minutes. By integrating the exercises into everyday life—such as after meals—they become part of a sustainable routine rather than an added burden. The quick, visible benefits (such as lower glucose readings) can also improve motivation and adherence over time [60,52]. Moreover, the exercises are adaptable to different physical abilities and health statuses, making them inclusive and widely accessible across diverse populations [6,66].
This study provides evidence that simple exercises, when performed with intention and structure, can deliver significant health benefits. The routine used—comprised of low-intensity leg lifts, trunk rotations, and arm movements—was sufficient to stimulate muscular activity and improve glucose regulation [50,10]. These movements activate large muscle groups, facilitating blood flow and encouraging glucose uptake [42,13]. Conducted two hours after eating, the timing helps counteract post-meal blood sugar surges [43,104. Performed for just ten to twelve minutes at an intensity of RPE 2–4, this approach offers a foundational exercise strategy that can be easily maintained [69,107]. It is especially suitable for beginners, older adults, or those with limited mobility, and can be progressively intensified as fitness improves [6,66].
The outcomes of this study align closely with global health initiatives, including the World Health Organization's Global Diabetes Compact, which promotes accessible, non-pharmacological interventions to combat the rising burden of diabetes diseases [1]. By demonstrating that short-duration physical activity can lead to substantial improvements in metabolic and cardiovascular markers, this study contributes to the growing body of evidence supporting lifestyle-based approaches to chronic disease prevention [6,52]. It offers a viable, low-cost method that can be deployed in community settings, healthcare environments, or home-based programs, reinforcing the message that effective diabetes management does not always require complex or resource-intensive interventions [16,108].
Conclusion
This study highlights the effectiveness of short, low-to-moderate intensity peripheral nervous system and musculoskeletal activation exercises in bioregenerative prevention and managing metabolic syndrome and promoting healthy aging. The exercises significantly reduced blood glucose and blood pressure, with minimal impact on heart rate, showing their potential for improving glycemic control and cardiovascular health. These simple, accessible exercises can be easily integrated into daily routines, offering a practical, low-cost solution for individuals, especially those with limited mobility or resources.
Future research should involve larger, more diverse groups, with longer interventions and deeper physiological measurements, to better understand the long-term benefits and optimal exercise protocols.
Overall, these findings support the use of peripheral nerves activation exercises as a viable strategy for managing metabolic syndrome and slowing aging, with the potential to enhance public health through simple, personalized bioregenerative exercise interventions.