Human Cytology: Classification and Quantitative Cell Landscape with Numbers, Types and Functional Diversity
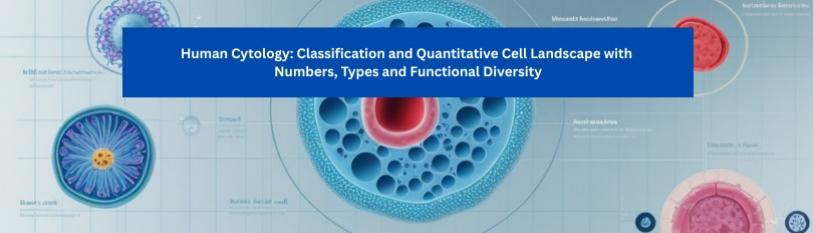
Mike KS Chan1,2,3,4,5,6*, Siti Azmah Jambo1,2,4, Mohd Iskandar Jumat1,2,4, Florisa Landa1,2,4, Nur Shafawati Saili1,2,4, Dianah Florentius1,2,4, Nuryasmin Ezzaty1,2,4, Raz Haziqah Hani Razali1,2,4, Brenda Song Pei Chui1,2,4, Aziera Farhanah Adihidayah Suardi1,2,4, Sze-Huey Sang1,2,4, Dmytro Klokol5,6, Olha Nishkumai5,6, Margarita Iemeliyanova5,6, Yuriy Nalapko5,6, Thomas Skutella7, Jonathan RT Lakey8,9, and Michelle BF Wong1,2,3,5
1Baden Research & Testing Laboratories Kota Kinabalu, Sabah, Malaysia
2EW Sanorell Research, Kota Kinabalu, Sabah, Malaysia
3Lincoln University College, Petaling Jaya, Selangor, Malaysia
4European Wellness Academy, Malaysia
5European Wellness Academy, Ukraine, Malaysia
6European Wellness Biomedical Group, Edenkoben Ukraine, Malaysia
7Institute for Anatomy and Cell Biology, III Medical Faculty, University of Heidelberg, Heidelberg, Germany
8University of California, Irvine- Department of Surgery, Irvine CA, USA
9University of California, Irvine- Department of Biomedical Engineering, Irvine CA, USA
*Corresponding author: Chan MKS, European Wellness Academy, Malaysia.
Citation: Chan MKS, Jambo SA, Jumat MI, Landa F, Saili NS, et al. Human Cytology: Classification and Quantitative Cell Landscape with Numbers, Types and Functional Diversity. J Stem Cell Res. 6(1):1-42.
Received: April 24, 2025 | Published: May 02, 2025
Copyright© 2025 genesis pub by Chan MKS et al. CC BY-NC-ND 4.0 DEED. This is an open-access article distributedunder the terms of the Creative Commons Attribution-NonCommercial-No Derivatives 4.0 International License.,This allows others distribute, remix, tweak, and build upon the work, even commercially, as long as they credit the authors for the original creation.
DOI: https://doi.org/10.52793/JSCR.2025.6(1)-71
Abstract
The human body is a masterpiece of biological engineering, consist of approximately 30 to 40 trillion cells. It represents a complex biological structure, with each cell type performing specialized roles essential for maintaining homeostasis. Despite decades of research, the precise counting and classification of these cells have long been hindered by outdated methods and incomplete datasets. Previous efforts, while foundational, often relied on oversimplified assumptions or generalized estimates and lacked of technological precision necessary for a comprehensive understanding. To fill this literature gap, this review offers a deeper, more refined exploration of human cell counts, delivering an integrated and insightful perspective. We trace the historical trajectory of cell count estimates, critically evaluating the progression, assumptions and limitations that shaped earlier works. Key seminal studies are revisited, focusing on how time and advancements in technologies have revolutionized our capacity to quantify and classify the diverse populations of human cells. Beyond simply presenting updated estimates, we also delve into the inherent variability in cell numbers and types, and addressing how certain factors influence cellular heterogeneity. Furthermore, we provide a crucial review of the different methodologies used to estimate cell counts, highlighting their strengths and weaknesses. Ultimately, we discuss the significance of precise cell counts in advancing various fields, positioning this review as an indispensable resource for future biomedical research. By addressing the limitations and gaps of previous studies, this review offers a more nuanced and precise understanding of human cellular diversity, establishing a new benchmark for the field.
Keywords
Human body; Human cell numbers; Cell count numbers; Cell types; Organs.
Introduction
Composed of an astonishing trillion cells, the human body functions as a highly complex and dynamic biological system, with each cell type carries out unique and essential roles vital for sustaining life. Determining the precise number of cells that make up the human body has been a scientific pursuit for centuries, offering profound insights into the fundamental workings of biology, medicine, and human health. Over the years, researchers have made remarkable progress in estimating these numbers. However, significant variability persists, primarily due to differences in assumptions, methodologies, and the complexity of cell diversity [1].
The discovery of the microscope in the 17th century paved the way for quantifying human cells for the first time. This innovative breakthrough signified the start of cellular biology and established the foundation for future advancements. Subsequent developments in imaging technologies, including electron and fluorescence microscope, combined with mathematical modeling, have facilitated more accurate estimates. Then, the introduction of stereological techniques and tissue-specific analyses has enabled scientists to refine these estimates more collectively [2-3]. Despite all of the significant technological advancements, limitations in resolving power and the ability to capture all cell types within complex tissue environments leave our understanding incomplete. Addressing these challenges will require even more refined tools and approaches to achieve a truly comprehensive view of human cellular composition.
Over and above that, previous research also has laid a comprehensive foundation for the study of human cell counts, contributing to methodological progressions, deepening our knowledge of cellular diversity, and highlighting the clinical relevance of accurate cell estimates. Unfortunately, differences in methodologies across studies lead to significant variability in estimated cell counts, making it difficult to compare the results and establish a universally accepted number of human cells [4].
Importantly, researchers found that cellular composition is far from uniform, it fluctuates throughout life, influenced by various factors such as age, sex, health conditions, and environmental influences. Many studies may not adequately account for these dynamics, leading to potentially misleading estimates. For example, the proportions of red blood cells, epithelial cells, and muscle cells can vary significantly across individuals and at different life stages [5-6]. Furthermore, lifestyle factors, including diet, nutrition, and physical activity, alongside genetic predispositions, contribute to the dynamic variation in cellular makeup. This highlights the complexity involved in estimating an accurate and universal cell count.
Understanding the total number of cells in the human body extends beyond mere academic curiosity, it also holds significant implications for medical research and clinical applications. Accurate estimates of cell counts are essential for advancing personalized medicine, which tailors treatments based on an individual's unique cellular composition [7]. Moreover, they play a pivotal role in diagnosing diseases related to cellular abnormalities, such as cancer and degenerative conditions, and in improving therapeutic strategies [8-9].
To address the current gaps, this review aims to offer an in-depth exploration of the evolution of human cell count estimates by highlighting the historical context, contributions of previous studies, and variability in the number of cells in the human body. We also address how cellular composition affect the cellular heterogeneity, accentuating on different technologies for cell count estimates and emphasizing the importance of precise cellular understanding in driving future advancements in both scientific research and medical innovation.
Historical Context: The Evolution of Cell Counts Estimates
In the past, scientists faced difficulty in determining the overall number of cells in the human body due to the wide range of cell sizes, types, and distribution throughout tissues. A closer look to the literature, early estimates were often speculative, relying on rudimentary methods based on average body mass and tissue volumes. Over time, more sophisticated methods were developed, yet there is still room left for further improvement. To recall, this historical overview outlines the origins of cellular research, emphasizing the key discoveries and approaches that have shaped our comprehension of cellular composition.
History of human cell
The growth of human cell exploration has been deeply impacted over the centuries by advances in microscopy, cell biology, and the wider knowledge of human physiology. The journey into human cells began with the development of microscope in the 17th century, which laid the foundation for modern cell biology (Figure 1).
During this period of transformation, significant contributions were made by trailblazers such as Robert Hooke and Antonie van Leeuwenhoek. Hooke's groundbreaking book Micrographia (1665) was innovative with its elaborate explanations and drawings of cells, bringing the idea of the cell to the scientific world. His detailed observations offered a fresh perspective on biological structures, laying the groundwork for future progress in cellular research [10].
Using refined lenses, Leeuwenhoek, who is commonly referred to as the 'father of microbiology', observed and documented a variety of cells, including blood cells and spermatozoa. His discoveries further enhanced the understanding of cellular diversity and complexity [11]. The contributions of Hooke and Leeuwenhoek sparked a new era in scientific discovery, advancing cell research towards uncharted territory.
Figure 1: The history of the microscope.
The Cell Theory
The mid-19th century saw the development of cell theory by scientists like Matthias Schleiden, Theodor Schwann, and Rudolf Virchow, which established a foundational basis for understanding cells. Schleiden and Schwann proposed that all living organisms are composed of cells, while Virchow famously stated that ‘Omnis cellula e cellula,’ meaning all cells arise from pre-existing cells [12]. This theory highlighted the significance of cells as the basic unit of life, leading researchers to concentrate on quantifying cells in various tissues and fluids. The theory rests on three main principles:
Human cell counts: from estimation to precision
The path to determining the number of cells in the body is being marked by a progression from rough estimates to more precise counts. The early 20th century saw significant inconsistencies in human cell count estimates due to the lack of advanced tools. However, as technology evolved, scientists unlocked more precise methods, revolutionizing our understanding of the human body's cellular makeup.
Current estimates
Initial estimates of the number of human cells varied widely, ranging from 10 to 100 trillion [18]. Recent study by Hatton et al. [19] have refined our understanding of the human cell count, suggesting that an average adult human is composed of approximately 36 trillion cells. This figure consists of a wide array of cell types, including red blood cells, neurons, epithelial cells, and more.
Significantly, the complex nature of human biology is also highlighted in this figure, where cell size, function, and lifespan vary widely across different tissues and organs. The researchers examine the variety of cell sizes, noting a broad range between the smallest cells, like erythrocytes, and the larger muscle cells. Cell size variability is crucial for tissue function, as each cell type plays a specific role based on its structure and composition. The human body relies on a mix of both small and large cells, working together to support essential biological processes.
The study's deeper significance lies in its focus on the factors that shape cell size distribution and overall cellular composition. This discovery has wide-ranging implications, not only for understanding basic human biology but also for advancing research in areas such as aging and disease. By demonstrating how changes in cell numbers and sizes affect our health, the study's insights could lead to new medical treatments. It highlights the value of studying cells to create better medical strategies tailored to each person's unique body.
Challenges in estimating cell counts
The complexity and variability in cellular composition make estimation of human cell counts a challenging task. The diversity of cell types, each with their own unique size, function, and turnover rate is one of the main technical hitches. For example, the lifespan and quantity of blood cells, epithelial cells, and neurons are significantly different. This variability makes it difficult to obtain a precise estimate across different tissues and organs. Furthermore, factors such as age, genetics, sex, and health conditions contribute to changes in cell counts, further complicating accurate estimates [2].
Another challenge lies in the dynamic nature of cellular turnover. Some cells, such as those in the skin and gut, constantly regenerate, while others, like neurons, remain largely static throughout life. According to Milo & Phillips [18], estimating the total number of cells requires accounting for both the steady-state populations and those undergoing continuous renewal. Advanced imaging and computational models have improved estimates, but variations between individuals still lead to uncertainties in the total count. Moreover, limitations in certain procedures, such as the difficulty of sampling certain tissues without causing damage, restrict our ability to make accurate counts. These factors together create a substantial challenge in determining an exact number of cells in the human body.
In short, the exploration of human cell counts offers profound insights into cellular biology and its medical significance. Although research has illuminated, current estimates reveal the complex nature of human cellular composition, underscoring the need for ongoing research to refine these numbers. As science progresses, the liaison between accurate cell counts and technological advancements will remain essential for improving health outcomes and addressing challenges related to aging and disease.
Key Studies and Their Contributions
The total number of cells in the human body is a key factor in understanding human biology, and disease development. However, estimating this number has been a difficult endeavor, marked by evolving methodologies, advances in technology, and increasing biological complexity. Over the years, several key studies have played a pivotal role in refining our knowledge in this area, each contributing new insights and assisting to build the foundation of our current understanding (Table 1). These studies highlight the complexity of cell distribution across various tissues and the inherent need for ongoing fine-tuning in cell number estimations.
Year | Main Topic | Number of Cells | Number of Cell Types | Number of Cell Groups | Key Findings | Reference |
1994 | Overview of cell biology, including cell types and functions | Not specified | ~200 | Multiple (not specified) | Comprehensive textbook covering cellular structure, function, and diversity in the human body. | [20] |
2002 | General overview of cell biology | Not specified | ~200 | Multiple (not specified) | Updated textbook edition, providing insights into cell diversity and function in the human body. | [21] |
2005 | General biology and human cell types | Not specified | ~200 | Multiple (not specified) | A biology textbook that discusses the diversity of cell types and their roles in human biology. | [22] |
2006 | Hematopoietic stem cells, cell count estimates | ~11,000-22,000 hematopoietic stem cells | 1 | 1 | Estimates the number of hematopoietic stem cells in humans and compares it across different mammals. | [23] |
2009 | Neuronal and non-neuronal cell counts in the human brain | ~86 billion neurons | ||||
~85 billion non-neuronal | 2 (neuronal, non-neuronal) | 1 (brain cells) | Estimates the number of neurons and non-neuronal cells in the human brain, emphasizing its complexity | [24] | ||
2009 | Neuronal and glial cells | ~86 billion neurons | ||||
~85 billion non-neuronal | 2 (neuronal, non-neuronal) | 1 (brain cells) | Estimated around 86 billion neurons and an approximately equal number of glial cells in the human brain. | [5] | ||
2012 | Neuronal scaling in the human brain | ~86 billion neurons | 2 (neuronal, non-neuronal) | 1 (brain cells) | Highlights the human brain as a scaled-up primate brain, showing proportional increases in neuron count with body size. | [25] |
2013 | Total cell number, cell types | ~37.2 trillion human cells | Not specified | Multiple (not specified) | Provided an estimate of 37.2 trillion human cells; detailed estimates for major cell types such as erythrocytes and muscle cells. | [6] |
2015 | Quantitative approach to cell biology | ~30 – 40 trillion human cells | ~200 | Multiple (not specified) | Provided detailed numbers for cells and cellular processes in various tissues. | [18] |
2016 | Total cell number | ~30 trillion human cells | Not specified | Multiple (not specified) | Revised estimate suggesting the number of human cells is around 30 trillion; bacterial cells in the body outnumber human cells. | [2] |
2017 | Human Cell Atlas project and single-cell sequencing | ~37 trillion human cells | ~200 | Multiple (not specified) | Describes the Human Cell Atlas project aimed at mapping all human cell types using single-cell sequencing. | [26] |
2019 | Spatial transcriptomics for tissue and cell type mapping | Not specified | Not specified | Multiple (not specified) | Introduces spatial transcriptomics for mapping cell types within tissues, enhancing understanding of tissue organization. | [27] |
2020 | Single-cell sequencing of human cells | Not specified | Not specified | Multiple (not specified) | Constructs a detailed single-cell map of human cells, highlighting cellular diversity. | [3] |
2023 | Immune cells in the human body | ~1.8 trillion immune cells | Not specified | 1 (immune cells) | Estimated the total mass, number, and distribution of immune cells, providing a refined understanding of the immune cell population in tissues. | [28] |
2023 | Human cell counts and size distribution | ~36 trillion human cells | ~400 | 1,264 separate cell groups | Provides a comprehensive review of the human cell count, including the size distribution and various types and groups. | [19] |
Table 1: Previous publications on the number of cells in the human body (1994-2023).
In the early studies from 1994 to 2005, most of the publications on cells are in the form of foundational textbooks that provide a general overview of cell biology without specific cell count estimates. Notable among them are works by Alberts et al. [20-21] and Campbell & Reece [22], which discuss cellular structure, function, and diversity in humans. They are fundamental resources for students and researchers, offering initial knowledge essential for further scientific inquiry and experimentation.
The research of Dingli & Pacheco in [23] specifically addresses the number of active hematopoietic stem cells (HSCs), estimating the count to be between 11,000 and 22,000. This study is trivial as it provides valuable insights into stem cell biology, particularly regarding the population of HSCs, which are crucial for blood formation and regeneration. The findings have important implications for medical fields such as bone marrow transplantation and the treatment of blood- related diseases, offering a clearer understanding of the stem cell pool available for these therapeutic interventions.
As time unfolds, research by Azevedo et al [24]. and Herculano-Houzel [5,25]. Have focused on the specialized topic of the human brain, particularly emphasizing the count of neuronal and non-neuronal cells. Azevedo et al [24]. Study estimating 86 billion and 85 billion of neuronal and non-neuronal cells respectively, emphasizing the isometric scaling of the human brain and its implications. Herculano-Houzel study in [5] corroborates the findings of Azevedo et al [24]. By also estimating similar numbers of neuronal and non-neuronal cells, highlighting the brain's complexity and its linear scaling compared to other primates. Meanwhile, the [25] publication delves further into this scaling, suggesting that the human brain is remarkably, yet not extraordinarily, different from other primates and discusses the evolutionary cost associated with such scaling.
Bianconi et al [6], directed one of the pioneering studies that delivered a comprehensive analysis of cell counts, arriving at an impressive estimate of approximately 37.2 trillion cells. They calculated this number by considering the volume and weight of various organs and tissues, each of which contains a unique distribution and concentration of cells. Their approach combined anatomical and physiological data by examining 53 cell types found in the human body and categorized them based on tissue and organ structures. The study also discusses the difficulties in achieving precise numbers due to variations among individuals. This estimation provides proper foundational data for related fields, helping in the study of diseases and therapeutic approaches at the cellular level.
Publication of book titled “Cell biology by the numbers” by Milo & Phillips in [18] estimated around 30-40 trillion of human cells, while Sender et al. [2] revised the total cell number to about 30 trillion, suggesting that bacterial cells in the body outnumber human cells. In 2017, Regev et al. [26] described the Human Cell Atlas project, aimed at mapping all human cell types using single-cell sequencing, which can significantly advance our knowledge of cell diversity and function, and potentially lead to breakthroughs in personalized medicine and disease treatment. Then in 2019, Vickovic et al. [27] introduced spatial transcriptomics for tissue and cell type mapping, enhancing our understanding of tissue organization, while Han et al. [3] constructed a detailed single-cell map of human cells, highlighting cellular diversity.
In 2023, Sender et al. [28] provide a sufficient census of immune cells in the human body, estimating that a 73 kg adult male has approximately 1.8 trillion immune cells. The study employs advanced methodologies to provide precise cell counts, contributing to improvement of techniques used in cell biology and related fields. By detailing the distribution and count of immune cells, the study enhances our knowledge of the immune system, which is vital for research in immunology.
A more recent study by Hatton et al. [19] provided a comprehensive review of human cell count and size distribution. This review presents a groundbreaking exploration of the size and number of cells in the human body. The study, which analyzed 1,264 distinct cell groups, comprising the 400 major cell types across 60 tissue types, estimated that human have around 36 trillion cells (29 trillion non-nucleated + 7 trillion nucleated cells) based on data from a reference male sample.
The studies over time show a progression from broader textbook overviews to specific, targeted research employing advanced methodologies like single-cell sequencing and spatial transcriptomics. This reflects growing precision in cell count estimates and an enhanced understanding of cell distribution and function. Collectively, these publications not only advance our knowledge of human cell numbers and distributions but also intersect significantly with practical and clinical applications, with implications for treating diseases and understanding human evolution. However, the existing research has many glitches in representing a consistent cell count in the human body. This issue remains insufficiently explored, and further studies are needed to fully understand the key aspects of cell counts.
Variation in Number of Cells and Types
The sheer variation in the numbers and types of cells underscores the complexity of human biology, showcasing the extraordinary specialization needed for different tissues and organs to carry out their distinct roles. The sophistication of our biological systems is highlighted by this cellular diversity, which also reflects the dynamic interaction needed to maintain health and homeostasis. The harmonious functioning of the body is achieved through the critical roles played by each cell type, which work in concert to make it an incredible marvel of nature.
Number of cells by types
Bianconi et al [6] study estimates the total number of cells in the average adult human body at around 37.2 trillion cells, but this number can vary depending on several factors such as age, sex, body composition, and size. This estimate was refined in a 2016 study by Sender et al. [2] which updated previous calculations and found that the human body contains approximately 30 trillion cells.
Then, seminal contributions have been made by Hatton et al. [19] which offer a more consistent and comprehensive quantitative framework of the cells in the human body. They build a hierarchical interface for the cellular organization of human body which compiles data from >1,500 published sources. Data on this website are intended to provide a holistic view of cell size, count and aggregate biomass of all the dominant cell types grouped into the tissues of the human body. Their reference samples include 70 kg man, 60 kg female and 10-year-old child (32 kg).
They estimate the total cell count to be approximately 36 trillion in males, 28 trillion in females, and 17 trillion in children. Cell counts are overwhelmingly dominated by red blood cells, platelets, and tissue-resident white blood cells, showcasing their vital roles in the body's function. An inverse relationship between cell size and cell count was also identified. This implies that while smaller cells like red blood cells dominate in number, larger cells like muscle fibers dominate in terms of biomass. The study suggests a trade-off between cell size and number across the body, indicating a form of cell-size regulation or homeostasis. Cells vary over seven orders of magnitude in size, from small red blood cells to large muscle fibers, and the distribution of cells across size classes follows a lognormal pattern.
Alternatively, it was reported in the literature that the human body consist of over 200 distinct types of cells, each specialized for specific functions. Data-derived from Sender et al. [2] highlights the dominance of red blood cells among the different cell types in the human body, which make up 84% of all cells. The data also showcasing the essential roles of less abundant but equally important cells like platelets, bone marrow cells, endothelial cells, and white blood cells each represent 2-5% of the total cell count. The "others" category includes various less abundant cell types that together account for 5%. This distribution emphasizes the crucial role of red blood cells in oxygen transport, while other cell types, though smaller in proportion, are essential for immune function, tissue repair, and overall health maintenance (Figure 2).
Figure 2: Distribution of the number of human cells by types.
Red blood cells
Red blood cells (RBCs), also known as erythrocytes, are the most abundant cells in the bloodstream, with each cubic millimeter of blood containing 4-6 million of these essential cells. RBCs' diameter of just 6 µm allows them to pass through even the tiniest blood vessels. The bone marrow generates 2- 3 million cells of RBCs every second, circulating tirelessly throughout the body for up to 120 days. When they become old or damaged, specialized macrophages in the spleen and liver remove them from circulation. Making up more than 80% of all human cells, RBCs are responsible for transporting oxygen and carbon dioxide through the circulatory system [2,19]. There are an estimated 20-25 trillion red blood cells in the human body.
Platelets
According to National Cancer Institute [29], platelets, also known as thrombocytes, are small, disc- shaped elements within the blood and spleen, derived from larger cells known as megakaryocytes in the bone marrow. They play a crucial role in blood clotting, helping to slow or stop bleeding and aiding in wound healing. In adults, a normal platelet counts falls between 150,000 and 450,000 platelets per microliter of blood [30]. A count below 150,000 platelets per microliter is considered lower than normal. Abnormalities in platelet count or function, whether an excess or deficiency can lead to health issues. Therefore, monitoring platelet levels in the blood is essential for diagnosing various diseases and conditions.
Bone marrow cells
Hidden within the core of most bones, lies bone marrow, a soft, spongy tissue teeming with blood vessels. This vital tissue comes in two forms, red and yellow [29]. Red bone marrow is a powerhouse, producing blood stem cells that transform into different types of blood cells. Meanwhile, yellow bone marrow, packed with fat, contains stem cells that have the ability to become cartilage, fat, or bone cells, fueling the body's growth and repair. Long regarded as the main hub for hematopoiesis (the production of blood cells), bone marrow is also a vibrant immune organ, playing a crucial role in regulating and mobilizing immune cells throughout the body [31].
Astonishingly, bone marrow is the birthplace and home for a remarkable 90% of the organism's total cells at any given moment, with anucleate RBCs and platelets representing the largest share of this cellular community [32]. Each day, the bone marrow produces an impressive number of cells, approximately 200 billion red cells, 10 billion white cells, and 400 billion platelets [33]. These vast quantities ensure the body maintains adequate levels of each cell type to perform essential functions such as oxygen transport, immune defense, and blood clotting.
Endothelial cells
Endothelial cells line the interior surface of blood vessels and form a cellular barrier that regulates the movement of macromolecules and cells into the subendothelial space of the arterial wall [21]. This barrier function is critical in maintaining vascular health by controlling the permeability of the vessel walls to various substances, including blood cells and plasma proteins [34]. The integrity of this endothelial layer is essential for preventing atherosclerosis and other vascular diseases, as it helps to prevent the buildup of plaque and the infiltration of potentially harmful substances into the walls of arteries [35]. The adult human body is home to at least one trillion endothelial cells, collectively weighing over 100 grams and spanning an impressive surface area of more than 3,000 square meters [36]. This enormous cell network connects and communicates with every other organ in the body, functioning as a distributed organ.
White blood cells
White blood cells (leukocytes) are immune cells, crucial components of the immune system, produced in the bone marrow and found throughout the blood and lymphatic tissues [37]. These include lymphocytes (T cells, B cells, natural killer (NK) cells), monocytes, neutrophils, eosinophils, and basophils. They play a pivotal role in defending the body against infections, diseases, and foreign invaders [38]. When they detect an infection or inflammation, they respond by mobilizing to the affected site to fight off invading pathogens or repair damaged tissue [39]. This response is essential for preserving health and stopping the body from becoming infected.
A vast army of specialized cells, each with a distinct function, fuel the immune system. T cells orchestrate and regulate immune responses, while B cells produce antibodies to neutralize invaders [40]. Meanwhile, macrophages act as the body's cleanup crew, engulfing and digesting cellular debris and microbes, while the primary mission of NK cells is to hunt down virus-infected cells and detect abnormal cells that could become or have already become tumor cells [41]. Rather than being restricted to one particular tissue, immune cells are found all around the body, where they are always on the lookout for dangers and preserving homeostasis. T cells, B cells, and dendritic cells concentrate in the lymphatic system, while 70% of plasma cells reside in the gut [28]. Neutrophils, eosinophils, monocytes, and basophils stay mostly in the bone marrow, with under 10% in the blood. Meanwhile, mast cells, NK cells, and macrophages are spread across tissues without a single dominant location.
Others (miscellaneous cells)
Beyond the more common cell types like red blood cells, the human body is home to a diverse array of specialized cells that perform essential but distinct roles. These "miscellaneous" cells, which include neurons, stem cells, and other types, support vital body processes including defense, communication, and tissue repair, enabling the body to function well under a variety of circumstances.
The basic building blocks of the nervous system are neurons, which produce and spread electrical impulses that carry signals throughout the body. Neurons power everything from conscious thought and memory to quick reflexes and smooth coordination, serving as the brain's vital communication network [42-43]. Many types of neurons share key traits, such as the presence of axons and dendrites, the ability to release vesicular transmitters, and the formation of pre- and post-synaptic connections, all essential for neural communication [44].
Glial cells, which are the predominant cells in the central nervous system, play a crucial role in brain function by supporting the health and operation of neurons. Out of these, oligodendrocytes, Schwann cells, astrocytes, microglia, and ependymal cells are highlighted as important contributors. Remarkably, most glial cells possess the ability to undergo mitotic division, making them essential for maintaining neural health [45].
Stem cells are undifferentiated cells and have the ability to transform into different types of cells as required by the body (Figure 3). They are responsible for driving tissue growth, repair, and regeneration. Found in both embryonic and adult tissues, these extraordinary cells have the unique ability to divide endlessly and transform into specialized cells like neurons, muscle cells, or blood cells, fueling the body’s natural healing and renewal processes [46].
Figure 3: The four different types of stem cells (Mike KS Chan, 2021 [47]).
Adipocytes, known as fat cells, have a broader range of functions beyond just storing energy. They also act as potent endocrine cells, emitting hormones that control metabolism and appetite and provide essential insulation and cushioning for the body. Though often associated with body fat, adipocytes are vital to maintain energy balance and play a key role in overall metabolic health [48].
The myocyte, also referred to as the muscle cell, is the tiniest component of every muscle tissue and organ in the body [49]. The human body contains three kinds of muscle cells: skeletal, smooth, and cardiac. Skeletal muscles are attached to bones to provide structure and strength to the body, cardiac muscle is found in the heart's walls and is responsible for pumping blood through the vasculature while smooth muscle can be located in various parts of the body such as blood vessels, GI tract, bronchioles, uterus, and bladder [50]. Muscle cells are generally large cells, ranging from 20 to 100 μm in diameter and several centimeters in length, with the longest fibers reaching around 12 cm. These cells have multiple nuclei in order to regulate the processes of protein synthesis and degradation [51].
Perceiving how the human body functions as a unified system relies heavily on understanding the distribution of cell types. Every kind of cell has specific functions in various organs, collaborating to uphold crucial processes such as circulation, respiration, and immune defense. This organization ensures the systems can communicate and support each other, allowing the body to regulate itself and respond to changes in the environment. Disruptions in the balance of cell types can lead to dysfunction and disease, emphasizing the importance of knowing how cells are distributed to fully understand the body's integrated and coordinated functions.
Number of cells by organ
Correspondingly, the human body is home to a wide array of vital organs, with the total number typically ranging from 78 to 80, depending on the definition of an "organ." The definition of an organ is not universally agreed upon, leading to varying numbers of organs depending on individual interpretations. The human body is equipped with well-known organs like the heart, lungs, liver, kidneys, and brain, along with lesser-known structures such as the mesentery and sensory organs. Each organ is made up of specialized cells that work in harmony to perform crucial functions. This diversity of organs adds to the complexity and precision of human physiology.
Figure 4: Number of cells by major organs of the human body.
Figure 4 visual illustrates the intricate distribution and variety of cells essential for the critical functions of major organs in the human body. The accompanying numbers represent the count of the primary cell types found in each organ which adapted from Mike KS Chan et al. [52] and Human Cell Treemap database [53]. The partitioning of the database results in 1,264 separate cell groups, representing the 400 major cell types across 60 organs/tissues. Table 2 presents the number of human cells by nine major organs in the human body, detailing the cell classes and cell types that support each organ's function.
Figure 5: The different types of lobes in the human brain [52].
The brain, as the body's control center, is populated by neurons and glial cells, neurons facilitate communication, while glial cells provide vital support and protection [54]. Korbinian Brodmann (1868–1918) has identified 52 different areas in the brain, called Brodmann areas (Figure 5). Each area has a special role, such as for movement, emotional control, or making decisions. Meanwhile, epithelial cells, macrophages, and endothelial cells collaborate in the lungs to facilitate gas exchange and immune responses, maintaining a continuous delivery of oxygen to the bloodstream.
The heart is primarily made up of cardiomyocytes (heart muscle cells), endothelial cells, and fibroblasts, all of which are crucial for the heart's pumping action and the maintenance of tissue integrity [55]. In the meantime, hepatocytes function as the primary liver cells, with important responsibilities in metabolism, detoxification, and bile synthesis. The stomach is lined with various cell types, including parietal cells, chief cells, and mucous cells, which are responsible for secreting digestive enzymes and acids [56].
The pancreas consists of exocrine cells, including acinar cells that produce digestive enzymes and ductal cells that secrete bicarbonate, as well as endocrine cells in the islets of Langerhans, which include alpha cells (producing glucagon), beta cells (producing insulin), delta cells (producing somatostatin), and PP cells (producing pancreatic polypeptide) [57]. A variety of cells, including nephrons, in the kidneys are essential for removing waste from the blood and regulating fluid levels. Finally, the small intestine contains epithelial cells, goblet cells, and enteroendocrine cells that aid in nutrient absorption and produce protective mucus, while the large intestine composed of colonocytes, goblet cells, and intestinal cells that focus on water absorption and the formation of solid waste [58].
In multicellular organisms like humans, successful body function depends on cell coordination, critical for achieving homeostasis, the required internal balance for survival. Cells need to consistently interact in order to control functions like growth, metabolism, immune responses, and reproduction. Cells do not function independently; they communicate with one another via cell signaling pathways. Chemical messengers like hormones, neurotransmitters, and cytokines are crucial for communication between cells, ensuring that they coordinate their activities to maintain homeostasis [59].
Together, these various types of cells form the tissues that make up organs. Organs then collaborate in systems such as the digestive, respiratory, cardiovascular, and nervous systems to ensure the survival of the organism. But then again, determining the number of cells distributed across different organs in the human body is challenging for several reasons such as diversity and complexity of cell types, organ-specific variability, limitations in sampling methods, technological constraints, and individual variability [2,19]. These challenges make it difficult to arrive at precise, standardized cell counts across all organs in the human body. There are key questions and notions that are still not discussed in the literature and previous research. The literature is useful in understanding the composition of the human body and can aid in numerous scientific and medical investigations, such as understanding disease mechanisms, developmental biology, and regenerative medicine.
Organ/Tissue: BRAIN | |||||||
Cell Classes | Type of Cells (Location) | ||||||
1 | Neuron | 1 | Betz Cells | ||||
2 | Von Economo neuron; Spindle cells | ||||||
3 | Hypocretin (orexin) cells | ||||||
4 | Vasopressin neurons | ||||||
5 | Corticothalamic neurons | ||||||
6 | Glucose-sensitive neurones | ||||||
7 | Glutamatergic relay cells | ||||||
8 | Cholinergic cells | ||||||
9 | Serotonergic neurons | ||||||
10 | AgRP NPY neurons | ||||||
11 | Catecholaminergic cells | ||||||
12 | Hippocampal-septal neurons | ||||||
13 | Medium spiny neurons | ||||||
14 | Hippocampal place cells | ||||||
15 | Isodendritic neurons | ||||||
16 | Purkinje cells | ||||||
17 | Fork cells | ||||||
18 | Dopaminergic cells | ||||||
19 | Magnocellular neurosecretory cells | ||||||
20 | Operational hub cells | ||||||
21 | Head direction cells | ||||||
22 | Grid cells | ||||||
23 | Mossy cells | ||||||
24 | Excitatory projection neurons | ||||||
25 | Hypothalamic CART neurons | ||||||
26 | Pyramidal tract neurons | ||||||
27 | Inferior olivary cells | ||||||
28 | Deep cerebellar nuclei neurons | ||||||
29 | Schaffer collateral associated neurons | ||||||
30 | Intratelencephalic neurons | ||||||
2. Interneuron | 31 | Interneurone specific cell 1 (IS-1) | |||||
32 | Cajal-Retzius cells | ||||||
33 | Martinotti cells | ||||||
34 | Double-bouquet cells | ||||||
35 | Unipolar brush cells | ||||||
36 | Cerebellar Golgi cells | ||||||
37 | Periglomerular cells | ||||||
38 | Candelabrum cells | ||||||
39 | Basket cells | ||||||
40 | Lugaro cells | ||||||
41 | Ivy cells | ||||||
42 | Neurogliaform cells | ||||||
43 | Cerebellar granule cells | ||||||
44 | Chandelier cells | ||||||
45 | Cortical interneurons | ||||||
46 | Rosehip cells | ||||||
47 | Superficial Short-Axon cells | ||||||
48 | Calretinin-expressing (CalP+) cells | ||||||
3. Stem cell | 49. Neural stem cells | ||||||
50. Perivascular mesenchymal stem cells | |||||||
4. Glial | 51 | Oligodendrocytes | |||||
52 | Tanycytes | ||||||
53 | Schwann cells | ||||||
54 | Astrocytes | ||||||
55 | Bergmann glial cells | ||||||
56 | Microglial; brain-resident macrophages | ||||||
57 | Leptomeningeal cells | ||||||
5. Precursor cell | 58 | Transient amplifying progenitors; C cells | |||||
59 | PSA-NCAM+ cells * | ||||||
60 | Neuroblasts | ||||||
61 | Ki67+ neural progenitor cells * | ||||||
62 | A2B5+ glial precursor cells * | ||||||
63 | White matter progenitor cells | ||||||
64 | NG2 cells; polydendrocytes | ||||||
65 | SOX2+ cells; Precursor cell with transcription factor * | ||||||
6. Specialized cell | 66. Human Brain Pericytes | ||||||
7. Support cell | 67. Perineural cells | ||||||
*Indicates cell of the human brain with surface marker or transcription factor | |||||||
Organ/Tissue: LUNG | |||||||
Cell Classes | Type of Cells (Location) | ||||||
1. Neuron | 1. C Postganglionic Motor Neuron; Cholinergic | ||||||
2. Endothelial | 2 | Bronchial Tissue Endothelial Cells | |||||
3 | Lungs Endothelial Cells | ||||||
3. Epithelial | 4 | Respiratory Ciliated Epithelial Cells (Bronchi) | |||||
5 | Respiratory Ciliated Epithelial Cells (Bronchioles) | ||||||
6 | Respiratory Preciliated Epithelial Cells (Trachea) | ||||||
7 | Bronchiole Clara Cell (Bronchial Tree) | ||||||
8 | Pulmonary Neuroendocrine Cell (Bronchial Tree) | ||||||
9 | Bronchi Indeterminate Cells | ||||||
10 | Bronchiole Indeterminate Cells | ||||||
11 | Alveolar Type 1 Pneumocyte | ||||||
12 | Alveolar Type 2 Pneumocyte | ||||||
13 | Goblet Cells (Bronchi) | ||||||
14 | Respiratory Serous Acinar Cells (Bronchial Tree) | ||||||
4. Stem cell | 15. Basal Epithelial Cells (Respiratory Bronchial) | ||||||
5. Lymphoid | 16 | Lymphocyte T-Cells; Cytotoxic (Bronchial Tree; LPLs) | |||||
17 | Lymphocyte T-Cells; Helper (Bronchial Tree; LPLs) | ||||||
18 | Lymphocyte T-Cells; Cytotoxic; IEL (Bronchial Tree) | ||||||
19 | Lymphocyte T-Cells; Helper; IEL (Bronchial Tree) | ||||||
20 | Lymphocyte T-Cells; Helper, memory; Pulmonary | ||||||
21 | Lymphocyte T-Cells; Cytotoxic, memory; Pulmonary | ||||||
22 | Lymphocyte T-Cells; Helper, naïve; Pulmonary | ||||||
23 | Lymphocyte T-Cells; Cytotoxic, naïve; Pulmonary | ||||||
24 | Lymphocyte B-Cells | ||||||
25 | NK Lymphocytes | ||||||
6. Fibroblast | 26. Fibroblasts (Aveolar Tissue) | ||||||
7. Pericyte | 27. Pericytes | ||||||
8. Other | nucleated | ||||||
blood | 28. Macrophages; Alveolar; Pulmonary | ||||||
9. Granulocyte | 29. Mast Cells; Pulmonary | ||||||
Organ/Tissue: HEART | |||||||
Cell Classes | Type of Cells (Location) | ||||||
1 | Cardiomyocyte | 1 | Cardiomyocytes | ||||
2 | Sinoatrial Node P-Cell | ||||||
3 | Sinoatrial Node Transitional Cells | ||||||
4 | Atrioventricular Node P-Cell (Ovid) | ||||||
5 | Atrioventricular Node (Rod Cells) | ||||||
6 | Bundle of His Cells | ||||||
7 | Heart Purkinje Fiber Cells | ||||||
2. Neuron | 8. C Postganglionic Motor Neuron; Cholinergic | ||||||
3. Endothelial | 9 | Blood Vessels | |||||
10 | Lymphatics | ||||||
11 | Endocardium | ||||||
4. Pericyte | 12. Pericytes | ||||||
5. White adipocyte | 13. White Adipocyte; medium & small | ||||||
6. Fibroblast | 14. Cardiofibroblast | ||||||
7. Epithelial | 15. Mesothelial Serous Epithelial Cells (Epicardium) | ||||||
16. Mesothelial Serous Epithelial Cells (Pericardium) | |||||||
8. Other | nucleated | ||||||
blood | 17. Macrophages | ||||||
9. Stem cell | 18. Telocyte | ||||||
Organ/Tissue: LIVER | |||||||
Cell Classes | Type of Cells (Location) | ||||||
1 | Epithelial | 1 | Hepatocytes | ||||
2 | Cholangiocytes; small | ||||||
3 | Cholangiocytes; medium | ||||||
4 | Cholangiocytes; large | ||||||
2. Endothelial | 5. Blood Vessels Endothelial Cells-f-Sinusoidal | ||||||
6. Lymphatics | |||||||
3. Pericyte | 7. Ito Cells | ||||||
4. Other | nucleated | ||||||
blood | 8 | Kupffer | |||||
9 | Monocytes | ||||||
5. Lymphoid | 10 | Pit Cells | |||||
11 | Lymphocyte NKT; IEL | ||||||
12 | Lymphocyte T-Cells; gamma delta; IEL | ||||||
13 | Lymphocyte T-Cell; Mixed; IEL | ||||||
6. White adipocyte | 14. White Adipocyte; medium & small | ||||||
7. Fibroblast | 15. Fibroblast | ||||||
Organ/Tissue: STOMACH | |||||||
Cell Classes | Type of Cells (Location) | ||||||
1 | Epithelial | 1 | Gastric Surface Mucous Epithelial Cells (Gastric Surface) | ||||
2 | Gastric Surface Mucous Epithelial Cells; Corpus, Fundic foveolae | ||||||
3 | Gastric Surface Mucous Epithelial Cells; Cardia foveolae | ||||||
4 | Gastric Surface Mucous Epithelial Cells; Antrum foveolae | ||||||
5 | Gastric Oxyntic Gland Neck Cells | ||||||
6 | Gastric Parietal Cell | ||||||
7 | Gastric Chief Cell | ||||||
8 | Gastric Antrum G-Cell | ||||||
9 | Gastric D-Cell | ||||||
10 | Enterochromaffin-Like Cell | ||||||
11 | Enterochromaffin; Serotonin | ||||||
12 | Gastric P/D1-Cell | ||||||
2. Smooth myocyte | 13. Gastro-Intestinal SMCs | ||||||
3. Other | nucleated | ||||||
blood | 14. Macrophages | ||||||
4. Granulocyte | 15. Mast Cells | ||||||
5. Lymphoid | 16. Lymphocyte T-Cell; Mixed; IEL | ||||||
6. Endothelial | 17. Blood Vessels (Stomach Endothelial Cells-f) | ||||||
18. Lymphatics (Stomach Endothelial Cells) | |||||||
7. Pericyte | 19. Pericytes | ||||||
8. Fibroblast | 20. Myofiboblast | ||||||
Organ/Tissue: PANCREAS | |||||||
Cell Classes | Type of Cells (Location) | ||||||
1 | Epithelial | 1 | Acinar Cells; Pancreatic; Mononuclear | ||||
2 | Acinar Cells; Pancreatic; Binuclear | ||||||
3 | Pancreatic Duct Centroacinar Cells | ||||||
4 | Pancreatic Duct Lining Intercalated Epithelial Cells | ||||||
5 | Pancreatic Duct Lining Intralobular Epithelial Cells | ||||||
6 | Pancreatic Duct Lining Interlobular 1 Epithelial Cells | ||||||
7 | Pancreatic Duct Lining Interlobular 2 Epithelial Cells | ||||||
8 | Pancreatic Duct of Wirsung Lining Interlobular Epithelial Cells | ||||||
9 | Pancreatic Duct of Santornini Lining Interlobular Epithelial Cells | ||||||
10 | Pancreatic Islet Alpha Cell | ||||||
11 | Pancreatic Islet beta cell | ||||||
12 | Pancreatic Enteroendocrine D-Cell | ||||||
13 | Pancreatic gamma cell | ||||||
14 | Pancreatic epsilon cell | ||||||
15 | Enterochromaffin; Serotonin | ||||||
2. Granulocyte | 16 | Mast Cells; Intralobular ducts | |||||
17 | Mast Cells; Interlobular ducts 1 | ||||||
18 | Mast Cells; Interlobular ducts 2 | ||||||
19 | Mast Cells; Duct of Wirsung | ||||||
20 | Mast Cells; Duct of Santorini | ||||||
3. Smooth myocyte | 21 | Pancreatic Interlobular Duct 1 SMCs | |||||
22 | Pancreatic Interlobular Duct 2 SMCs | ||||||
23 | Pancreatic Duct of Wirsung SMCs | ||||||
24 | Pancreatic Duct of Santorini SMCs | ||||||
4. Stem cell | 25. Interstitial Cell of Cajal | ||||||
5. White adipocyte | 26. White Adipocyte; very small | ||||||
6. Fibroblast | 27. Fibroblasts | ||||||
7. Endothelial | 28 | Pancreas Exocrine Capillaries Endothelial Cells | |||||
29 | Pancreas Endocrine Blood Vessels Endothelial Cells-f | ||||||
30 | Lymphatics | ||||||
8. Pericyte | 31. Pericytes (Pancreas Exocrine) | ||||||
32. Pericytes (Pancreas Islets) | |||||||
9. Other | nucleated blood | 33. Macrophages | |||||
Organ/Tissue: KIDNEY | |||||||
Cell Classes | Type of Cells (Location) | ||||||
1 | Endothelial | 1 | Blood Vessels Endothelial Cells-f (Kidney Cortical Glomeruli) | ||||
2 | Blood Vessels Endothelial Cells-fd (Kidney Cortical Peritubular) | ||||||
3 | Lymphatics (Kidney Cortical Endothelial Cells) | ||||||
4 | Kidney Medullary Descending Vasa Recta tDL Endothelial Cells | ||||||
5 | Kidney Medullary Asc. Vasa Rcta tAL Blood Vessels Endothelial Cells-fd | ||||||
6 | Kidney | Cortex-Medulla | TAL | Vasa | Rcta | Blood | Vessels |
Endothelial Cells-fd | |||||||
2. Epithelial | 7 | Glomerular Parietal Epithelial Cells | |||||
8 | Glomerular Podocytes | ||||||
9 | Proximal Tubule S1 Epithelial Cells (Kidney Cortex) | ||||||
10 | Proximal Tubule S2 Epithelial Cells (Kidney Cortex) | ||||||
11 | Proximal Tubule S3 Epithelial Cells (Kidney Medullary) | ||||||
12 | Descending Thin Loop Of Henle; DtL Type-1 Epithelial Cells | ||||||
13 | Descending Thin Loop Of Henle; DtL Type-2 Epithelial Cells | ||||||
14 | Descending Thin Loop Of Henle; DtL Type-3 Epithelial Cells | ||||||
15 | Ascending Thin Loop Of Henle; AtL Type-4 Epithelial Cells | ||||||
16 | Thick Ascending Loop; Medullary; M-TAL Epithelial Cells | ||||||
17 | Thick Ascending Loop Of Henle; Cortex; C-TAL Epithelial Cells | ||||||
18 | Macula Densa Cell | ||||||
19 | Juxtaglomerular Cell | ||||||
20 | Distal Convoluted Tubule Epithelial Cells | ||||||
21 | Connecting Tubule Principal Cell | ||||||
22 | Connecting Tubule Intercalated A Cell | ||||||
23 | Connecting Tubule Intercalated B Cell | ||||||
24 | Cortical Collecting Principal Cell | ||||||
25 | Cortical Collecting Intercalated A Cell | ||||||
26 | Cortical Collecting Intercalated B Cell | ||||||
27 | Outer Medullary Collecting Tubule Principal Cell | ||||||
28 | Collecting Tubule Intercalated A Cell | ||||||
29 | Collecting Tubule Intercalated B Cell | ||||||
30 | Inner Medullary Collecting Tubule Principal Cell | ||||||
31 | Urothelium Umbrella Cells (Kidney minor calyces) | ||||||
32 | Urothelium Intermediate Cells (Kidney minor calyces) | ||||||
33 | Urothelium Basal Cells (Kidney minor calyces) | ||||||
34 | Urothelium Umbrella Cells (Kidney major calyces) | ||||||
35 | Urothelium Intermediate Cells (Kidney major calyces) | ||||||
36 | Urothelium Basal Cells (Kidney major calyces) | ||||||
3. Pericyte | 37 | Intraglomerular Mesangial Cells | |||||
38 | Extraglomerular Mesangial Cells | ||||||
39 | Pericytes (Kidneys Cortex) | ||||||
40 | Pericytes (Kidneys Medullary) | ||||||
4. Smooth myocyte | 41. Papillary Duct, Calyx, and Pelvis SMCs | ||||||
42. Capsule Inferior Layer SMC | |||||||
5. Stem cell | 43. Interstitial Cell of Cajal | ||||||
6. Fibroblast | 44 | Fibroblasts (Kidney Capsule) | |||||
45 | Fibroblasts (Kidney Cortical) | ||||||
46 | Fibroblasts (Kidney Medullary) | ||||||
7. White adipocyte | 47. White Adipocyte; very small | ||||||
8. Other nucleated blood | 48 | Dendritic Cells (Kidneys Cortical) | |||||
49 | Dendritic Cells (Kidneys Medullary) | ||||||
50 | Macrophages (Kidneys Cortical) | ||||||
51 | Macrophages (Kidneys Medullary) | ||||||
9. Lymphoid | 52. Lymphocyte T-Cell; Mixed (Kidneys Cortical) | ||||||
53. Lymphocyte T-Cell; Mixed (Kidneys Medullary) | |||||||
54. Lymphocyte B-Cells (Kidneys Cortical) | |||||||
55. Lymphocyte B-Cells (Kidneys Medullary) | |||||||
10. Granulocyte | 56. Neutrophils | ||||||
Organ/Tissue: SMALL INTESTINE | |||||||
Cell Classes | Type of Cells (Location) | ||||||
1 | Epithelial | 1 | Enterocytes; Villa Epithelial Cells | ||||
2 | Enterocytes; Crypt Epithelial Cells | ||||||
3 | Goblet Cells | ||||||
4 | Paneth Cell | ||||||
5 | Enteroendocrine Cell | ||||||
6 | Enterochromaffin; Serotonin | ||||||
7 | Intestinal D-Cell | ||||||
8 | Intestinal I-Cell | ||||||
9 | Intestinal L-Cell | ||||||
10 | Intestinal K-Cell | ||||||
11 | Intestinal M-Cell | ||||||
12 | Intestinal Tuft-Cell | ||||||
13 | Transit-Amplifying Epithelial Cells | ||||||
14 | Mesothelial Serous Epithelial Cells (Gastrointestinal Tract) | ||||||
2. Stem cell | 15. Crypt Stem Cells | ||||||
16. Interstitial Cell of Cajal (Gastrointestinal Tract) | |||||||
3. Other | nucleated | ||||||
blood | 17. Macrophages | ||||||
4. Granulocyte | 18. Eosinophils (Gastrointestinal Tract) | ||||||
19. Mast Cells | |||||||
5. Lymphoid | 20 | Lymphocyte T-Cell; Mixed; Sm Intest (Lamina Propria) | |||||
21 | Lymphocyte B-Cells; Sm Intest (Lamina Propria) | ||||||
22 | Lymphocyte T-Cell; Mixed; Sm Intest (Peyer's Patches) | ||||||
23 | Lymphocyte B-Cells; Sm Intest (Peyer's Patches) | ||||||
24 | NK Lymphocytes; IEL | ||||||
25 | Lymphocyte T-Cells; Helper; IEL | ||||||
26 | Lymphocyte T-Cells; Cytotoxic; IEL | ||||||
27 | Lymphocyte T-Cells; gamma delta; IEL | ||||||
28 | Plasma Cells | ||||||
6. Smooth myocyte | 29. Gastro-Intestinal SMCs | ||||||
7. Endothelial | 30. Blood Vessels | ||||||
31. Lymphatics | |||||||
8. Pericyte | 32. Pericytes | ||||||
9. Fibroblast | 33. Myofibroblasts | ||||||
54. Lymphocyte B-Cells (Kidneys Cortical) | |||||||
55. Lymphocyte B-Cells (Kidneys Medullary) | |||||||
10. Granulocyte | 56. Neutrophils | ||||||
Organ/Tissue: SMALL INTESTINE | |||||||
Cell Classes | Type of Cells (Location) | ||||||
2 | Epithelial | 15 | Enterocytes; Villa Epithelial Cells | ||||
16 | Enterocytes; Crypt Epithelial Cells | ||||||
17 | Goblet Cells | ||||||
18 | Paneth Cell | ||||||
19 | Enteroendocrine Cell | ||||||
20 | Enterochromaffin; Serotonin | ||||||
21 | Intestinal D-Cell | ||||||
22 | Intestinal I-Cell | ||||||
23 | Intestinal L-Cell | ||||||
24 | Intestinal K-Cell | ||||||
25 | Intestinal M-Cell | ||||||
26 | Intestinal Tuft-Cell | ||||||
27 | Transit-Amplifying Epithelial Cells | ||||||
28 | Mesothelial Serous Epithelial Cells (Gastrointestinal Tract) | ||||||
2. Stem cell | 15. Crypt Stem Cells | ||||||
16. Interstitial Cell of Cajal (Gastrointestinal Tract) | |||||||
3. Other | nucleated | ||||||
blood | 17. Macrophages | ||||||
4. Granulocyte | 18. Eosinophils (Gastrointestinal Tract) | ||||||
19. Mast Cells | |||||||
5. Lymphoid | 20. Lymphocyte T-Cell; Mixed; Sm Intest (Lamina Propria) | ||||||
21 | Lymphocyte B-Cells; Sm Intest (Lamina Propria) | ||||||
22 | Lymphocyte T-Cell; Mixed; Sm Intest (Peyer's Patches) | ||||||
23 | Lymphocyte B-Cells; Sm Intest (Peyer's Patches) | ||||||
24 | NK Lymphocytes; IEL | ||||||
25 | Lymphocyte T-Cells; Helper; IEL | ||||||
26 | Lymphocyte T-Cells; Cytotoxic; IEL | ||||||
27 | Lymphocyte T-Cells; gamma delta; IEL | ||||||
28 | Plasma Cells | ||||||
6. Smooth myocyte | 29. Gastro-Intestinal SMCs | ||||||
7. Endothelial | 30. Blood Vessels | ||||||
31. Lymphatics | |||||||
8. Pericyte | 32. Pericytes | ||||||
9. Fibroblast | 33. Myofibroblasts | ||||||
Organ/Tissue: LARGE INTESTINE | |||||||
Cell Classes | Type of Cells (Location) | ||||||
1 | Epithelial | 1 | Colonocytes; Surface | ||||
2 | Colonocytes; Crypt | ||||||
3 | Enterochromaffin; Serotonin | ||||||
4 | Intestinal D-Cell | ||||||
5 | Intestinal I-Cell | ||||||
6 | Intestinal L-Cell | ||||||
7 | Intestinal K-Cell | ||||||
8 | Intestinal Tuft-Cell | ||||||
9 | Goblet Cells | ||||||
2. Stem cell | 10. Crypt Stem Cells | ||||||
3. Other | nucleated | ||||||
blood | 11. Macrophages | ||||||
12. Dendritic Cells | |||||||
4. Lymphoid | 13 | Lymphocyte B-Cells | |||||
14 | NK Lymphocytes; IEL | ||||||
15 | Lymphocyte T-Cells; Helper; IEL | ||||||
16 | Lymphocyte T-Cells; Cytotoxic; IEL | ||||||
17 | Lymphocyte T-Cells; gamma delta; IEL | ||||||
18 | Plasma Cells | ||||||
5. Smooth myocyte | 19. Gastro-Intestinal SMCs | ||||||
6. Endothelial | 20. Blood Vessels | ||||||
21. Lymphatics | |||||||
7. Pericyte | 22. Pericytes | ||||||
8. Fibroblast | 23. Myofibroblasts | ||||||
9. Granulocyte | 24. Mast Cells |
Factors Influencing Cellular Composition
The makeup of cells in the human body is always changing due to various factors that constantly interact over a person's lifetime. These aspects include biological factors like age, genetics, and sex, as well as lifestyle choices such as diet, physical activity, and environmental factors. Comprehensively appreciating how cellular composition evolves and impacts overall health is essential by understanding these interconnected factors.
Aging
Aging affects a variety of cellular processes, resulting in decreased function of stem cells, decreased efficiency of the immune system, changes in muscle makeup, and alterations in adipose tissue metabolism. "Chronological aging" and "replicative aging" contribute to cellular damage, particularly in continuously dividing cells like those in the adult stem cells, as well as in the skin or gut. This damage increases the risk of apoptosis, cellular senescence, and malignant transformations [60-61].
On the other hand, aging impacts immune cell composition, leading to a decline in T and B lymphocyte numbers and function, weakening immune responses and increasing susceptibility to infections, cancer, and autoimmune diseases [62-63]. The composition of muscles is greatly impacted by aging, leading to sarcopenia, which is characterized by a reduction in muscle mass and fiber size, especially in fast-twitch fibers. This leads to decreased strength, mobility, and an increased risk of falls in older adults [64-65]. Lastly, aging alters adipose tissue, causing hypertrophy and inflammation, which contribute to metabolic dysfunction, obesity, and related complications [66-67].
Genetics
Genetics plays a crucial role in shaping cellular composition and function, with research highlighting the impact of gene expression, genetic disorders, environmental interactions, and aging on health outcomes. Duddu, Chakrabarti, Ghosh, and Shukla [68] and Breschi et al. [69] emphasize the importance of transcription factors and core transcriptional networks in regulating the differentiation and function of major cell types, including those in hematopoietic and cardiovascular systems. Genetic variations in these factors can lead to altered cellular behavior, contributing to cardiovascular diseases, cancer, and genetic disorders.
Genetic mutations significantly influence disease development, as demonstrated by Jackson, Marks, May, and Wilson [70], who categorize diseases into single-gene disorders, chromosomal abnormalities, and multifactorial patterns. Mutations in key genes, such as those related to cancer, disrupt normal cellular processes like DNA repair and cell signaling, leading to uncontrolled growth. Bobadilla, Macek, Fine, and Farrell [71] highlight CFTR mutations in cystic fibrosis, that impair cellular function, particularly in ion transport and fluid balance, causing health complications.
Environmental factors also interact with genetics to shape immune responses and cellular environments, as described by Virolainen, VonHandorf, Viel, Weirauch, and Kottyan [72]. This interaction influences cell functions such as programmed cell death and cell growth, leading to conditions like autoimmune diseases, cancers, and metabolic disorders. Chandrasekaran & Weiskirchen [73] explore how genetic predispositions influence fat cell distribution and metabolic pathways, contributing to insulin resistance and type 2 diabetes mellitus. Last of all, Rodríguez-Rodero et al. [74] discuss how genetics influences aging, highlighting that epigenetic modification and gene expression changes alter cellular function and composition over time. These genetic factors play a role in causing variations in phenotypes associated with aging and in increasing susceptibility to age-related diseases.
Biological differences between sexes
Sexes significantly impact cellular composition, function, and health outcomes. X-inactivation in females results in a mosaic expression of X-linked genes, leading to diverse cellular phenotypes in contrast to males, who have only one X chromosome. This variability affects immune response, metabolism, and disease susceptibility [75]. Inherent genetic and hormonal differences between males and females contribute to distinct health outcomes, with males being more prone to heart disease and females showing stronger immune responses but higher susceptibility to autoimmune diseases [76-77].
Variations in metabolism can be seen, as males generally have higher muscle mass and lower fat mass, impacting energy usage during long periods of physical exertion [78]. Hormone imbalances caused by obesity can disturb the function of fat cells, impacting how fats are processed and raising the chance of heart disease [79]. Furthermore, brain development and behavior are influenced by sex hormones, leading to cognitive and emotional variations between the sexes, particularly in individuals with disorders of sex development [80-82].
Diet and nutrition
Diet and nutrition are essential for cellular function, makeup, and general well-being. Research by Leidy et al. [83] and Westerterp-Plantenga, Lemmens, and Westerterp [84] stress the importance of sufficient protein in the diet for maintaining weight and promoting cellular health, which improves overall function and regeneration. Diet also influences stem cell behavior and differentiation. In their study conducted in 2022, Puca, Fedele, Rasio, and Battista [85] discovered that stem cell dynamics are influenced by both macro- and micronutrients. They observed that a well-balanced diet supports optimal stem cell function, whereas unhealthy dietary habits may accelerate cancer development.
Next, Valdes, Walter, Segal, and Spector [86] emphasize that dietary patterns rich in diverse nutrients positively affect gut health and immune responses. A healthy gut microbiome, supported by a diet rich in fiber and plant-based foods, enhances cellular health and metabolic processes, while imbalances can lead to inflammatory diseases [87-88]. Micronutrients are vital for immune function, especially as the body ages, their deficiency can impair immune responses and disrupt cellular dynamics [89].
An imbalance between the production of reactive oxygen species (ROS) and their detoxification can cause oxidative stress, which is linked to cell damage, long-lasting inflammation, and diseases like cancer [90]. Additionally, protein consumption is essential for repairing muscles and maintaining good metabolic health, especially for people who are active [91]. Furthermore, ensuring the plasma membrane's integrity is vital for cellular well-being, since any disturbances can result in conditions like cancer and neurodegenerative disorders [92].
Physical activities
Physical activity plays a crucial role in shaping cellular composition and function across various tissues. Hawley, Hargreaves, Joyner, and Zierath [93] explain that regular exercise boosts muscle metabolism, promotes mitochondrial biogenesis, and promotes muscle growth. It also strengthens the immune system by improving immune cell function. In addition, exercise significantly enhances mucosal immunity, as noted by Bishop and Gleeson [94], who found that both acute and chronic physical activity increase essential immune markers like immunoglobulins and cytokines. This not only boosts the immune response but also improves the function of immune cells, leading to lower infection risk and better overall cellular health.
Kolnes, Peterson, Lien-Iversen, Højlund, and Jjensen [95] investigate how exercise affects the composition of adipose tissue, revealing that physical activity improves the cellular structure of adipose tissue, leading to better fat storage and decreased inflammation. This change results in increased sensitivity of fat cells and better body structure, demonstrating how lifestyle modifications can boost metabolic well-being and combat obesity-related issues.
Furthermore, Carter and Hinton [96] emphasize the significance of physical activity in maintaining bone health by explaining how weight-bearing exercises help to stimulate bone formation and remodeling, essential for preserving bone density and strength. Regular physical activity encourages the growth and specialization of bone-building cells, leading to enhanced bone strength, especially among individuals vulnerable to osteoporosis and fractures.
Environmental factors
Pollutants have a strong influence on immune cell function, cellular health, and disease progression in the body due to environmental factors. Research conducted by Glencross, Ho, Camiña, Hawrylowicz, and Pfeffer [97] and Ural et al. [98] demonstrates that air pollution, including particulate matter and gases, can hinder immune reactions, resulting in enhanced inflammation and greater vulnerability to infections. Furthermore, Rahman, Rahman, Parvez, and Kim [99] elaborate on the impact of environmental toxins on autophagy, an essential cellular function that breaks down and recycles cellular parts. Being exposed to contaminants such as heavy metals can disrupt the regulation of autophagy, causing the buildup of faulty organelles and proteins, which can lead to cellular stress, inflammation, and cell death.
Chinta et al. [100] conducted research on the neurotoxin paraquat and discovered its involvement in the aging process of neurons, particularly in connection to Parkinson's disease. Exposure to paraquat accelerates aging in neuronal cells, manifesting in altered shape and increased release of pro-inflammatory cytokines. This procedure results in neurodegeneration, showing how external factors can initiate cell aging and impact brain well-being. Baccarelli and Bollati [101] point out that environmental exposure can result in epigenetic modifications, where chemicals can alter gene expression without changing the DNA sequence. Modifications like DNA methylation and changes to histones have a significant effect on how cells function and can play a role in diseases like cancer.
Finally, Thompson et al. [102] state that cancer risk increases due to environmental immune disruptors, which cause chronic inflammation and changes in cellular functions. This ongoing inflammation can harm cell structures, interfere with communication pathways, and cause changes in DNA, ultimately resulting in the start and development of cancer.
Health conditions
Cellular interactions and dysfunction have a significant impact on health conditions like cancer, cardiovascular disease (CVD), chronic inflammation, atherosclerosis, and systemic lupus erythematosus (SLE). The importance of the tumor microenvironment (TME) in cancer advancement was emphasized by Anderson and Simon [103]. The TME is made up of various types of cells, such as cancer and immune cells, that work together to promote tumor growth, evade the immune system, and induce metabolic alterations. Having an awareness of this is crucial for creating successful cancer therapies, as the TME plays an active role in tumor activity [104].
In CVD, Martins-Marques, Coutinho, and Kiss [105] emphasize that the dysfunction of endothelial cells and cardiomyocytes play a crucial role in the advancement of the disease. Henein, Vancheri, Longo, and Vancheri (2022) [106] found that chronic inflammation, characterized by heightened inflammatory markers and compromised immune responses, worsen cardiovascular disease by perpetuating a cycle of inflammation that impacts T cell populations.
Rohm, Meier, Olefsky, and Donath [107] examine the connection between persistent inflammation, which is frequently associated with obesity, and the interference in insulin signaling, as well as the heightened vulnerability to diabetes and CVD. Senescent cells continue to promote inflammation, weakening immune function and enhancing susceptibility to infections. Long-lasting inflammation can also lead to tissue scarring and genetic changes, which are linked to the onset of cancer [62].
Atherosclerosis is characterized by the accumulation of fatty deposits and cellular waste in arteries, leading to damage of the endothelium, inflammation, and an increase in smooth muscle cell growth [108]. Inflammation worsens with immune cell infiltration and impacts cellular makeup, whereas vascular smooth muscle cells (VSMCs) are crucial in forming and maintaining plaques [109].
SLE, a challenging autoimmune disorder, is marked by immune cell irregularities [110]. Alterations in cell death and immune cell behavior play a role in prolonged inflammation and autoimmune diseases, underscoring the importance of specific treatments [111-112]. Additionally, changes in B cell overactivity and T cell performance have a significant effect on the development of SLE [113- 114].
To sum up, the factors mentioned earlier are crucial in determining cellular makeup and add to the ever-changing characteristics of cell populations. Biological factors such as age, genetics, and sex have significant impacts on the development of cellular diversity, which can be further altered by lifestyle decisions such as diet and physical activity, as well as environmental factors. Understanding health and disease requires recognizing how the cellular composition can change, highlighting the necessity of taking a holistic approach to wellness. These different factors allow us to gain a deeper understanding of how they impact the complexity of human biology and guide approaches for enhancing health outcomes.
Methods for Estimating the Number of Cells in the Human Body
Estimating the overall number of cells in the human body is a difficult task, requiring a mix of different methods to accommodate the wide range of cell sizes, structures, and roles. Precise estimation of cell count is essential in developmental biology, aging research, and tissue engineering. This section of the review gives a summary of the main methods employed to calculate cell numbers in the human body, with a focus on stereological techniques, mathematical modeling, and tissue- specific cell counting.
Stereological techniques
Stereology represents a quantitative framework for sampling and analyzing biological material to infer geometric properties, such as number, length, volume, and surface area, from three- dimensional (3D) structures [115]. This approach has been widely used in biological tissue analysis, including estimating the number of neurons within specific brain regions, the length of capillaries, the volume of tumor cells, and the surface area of membranes. Stereological methods, such as the dissector method, point counting, nucleator method, and optical fractionator, are frequently employed to infer 3D properties from 2D histological sections. Notably, the optical fractionator has been the standard for neural cell counting over the past two decades, particularly in neurogenesis studies [116].
Although stereological techniques are considered unbiased and efficient for tissue-level cell counting, their accuracy is contingent upon the quality of histological samples and the expertise of personnel conducting the analysis. Furthermore, these methods rely on assumptions of tissue homogeneity, which may not hold in organs with complex architectures, such as the brain or liver, where significant regional variations can complicate the uniform application of stereological models.
Mathematical modeling
Mathematical modeling provides an alternative method for estimating total cell counts by integrating data from various tissues and organs. This approach typically involves estimating the number of cells in a single organ or tissue (e.g., the skin, liver, or brain) and extrapolating to the entire body. By applying this methodology across different tissue types such as epithelial cells, muscle cells, and neurons, researchers can develop comprehensive estimates of total cell numbers.
One prominent study by Bianconi et al. [6] estimated that the human body contains approximately 37.2 trillion cells by synthesizing data from multiple tissues. However, challenges arise due to tissue heterogeneity and dynamic physiological changes, complicating the extrapolation process. As a result, the accuracy of mathematical models is closely tied to the quality of empirical data derived from histological imaging and physiological studies, highlighting the need for high- quality, tissue-specific data to refine these estimates.
Tissue-specific cell counts
Tissue-specific cell counting offers a method to provide more precise estimations by accounting for the structure, function, and composition of individual tissues. This approach can improve the accuracy of cell counts, particularly when integrated with other methods. For instance, a recent study by Conde et al. [117] introduced CellTypist, a machine learning tool designed to rapidly and accurately annotate immune cell types, addressing the complexity of the immune system. This system allows researchers to assess the diversity of immune cells distributed throughout the body, which collectively maintain tissue homeostasis and mediate immunity.
In contrast, blood cell counting is often performed using automated hematology analyzers, which determine the concentration of erythrocytes, leukocytes, and platelets per microliter of blood [118]. These values, when multiplied by total blood volume, provide an estimate of overall blood cell count. Additionally, methods such as immunohistochemistry, which identifies specific cell types, are frequently combined with stereological approaches like the optical fractionator to estimate the number of neurons and glial cells in rodent models [119].
For tissues like the skin, where cells are organized into distinct layers, cell counts are typically calculated by measuring surface area and estimating the number of cells per unit area [120]. By combining this with stereological methods, researchers can generate highly accurate estimates of epithelial cell numbers. Tissue-specific techniques are becoming increasingly indispensable with advancements in automation and machine learning, which improve both speed and precision.
In summary, while each method for estimating cell counts, whether stereological, mathematical, or tissue-specific has its own strengths and limitations, a combination of approaches often yields the most reliable results. The accuracy of these methods largely depends on the quality of the underlying data, the assumptions about tissue properties, and the technological advancements that facilitate more precise cell counting. As research progresses, integrating modern computational tools with established biological techniques will likely enhance our ability to estimate the number of cells in the human body with greater precision.
Significance of Understanding Cell Counts in Medicine
The number and distribution of cells in the human body hold significant implications for medical research and clinical practice. Cell counting, in particular, plays a vital role in the early diagnosis of cancer and other disorders like breast cancer, anemia and Alzheimer’s disease. Plus, procedures such as stem cell therapy, which aim to boost cell numbers, are growing in importance within contemporary medicine, as they aid in replacing injured cells and improving overall treatment results.
Cancer
The number of cells is an essential factor in diagnosing cancer, as unregulated cell growth is a significant sign of cancer development [121]. Cancer cells usually show irregular proliferation and prolong their lifespan beyond the regular cell growth period. In contrast, normal cells maintain a well-regulated cycle of growth and survival. In a cancerous state, normal cells gradually transform into a neoplastic state, invading surrounding tissues and metastasize to other parts of body. This process drives the multistep development of tumor formation, where abnormal cell proliferation happens [16]. Cell counting can detect these over-proliferating cells and is therefore a key method in diagnosis of diseases, while guiding therapeutic decisions.
Conventional diagnostic methods, such as low dose computed tomography (CT) scans are limited by high cost and invasive radiation exposure, while tissue biopsies are invasive, painful and challenging to operate depending on the tumor site [122]. In contrast, liquid biopsy provides a less invasive and affordable option, not just for early cancer detection but also for predicting outcomes, assessing changes over time, and identifying molecular characteristics [123]. This method involves examining blood samples to identify biomarkers such as circulating tumor cells (CTCs) and circulating tumor DNA (ctDNA) [123-124]. It is particularly beneficial for identifying cancer types such as breast, prostate, and non-small cell lung cancer [123-125].
On the other hands, utilizing microfluidic cell technology to digitally measure circulating tumor cells (CTCs) offers essential prognostic data in prostate cancer according to Sparano et al. (2018) [126]. Basically, CTCs play a key role in metastasis by coming from the primary tumor, moving through the bloodstream, and spreading to other organs, ultimately causing the growth of secondary tumors [127]. Therefore, CTCs are considered promising biomarkers for early cancer detection and dynamic evaluation. Through measurement of CTCs, cancer progression outcomes like late clinical recurrence can be predicted, accommodating in guiding clinicians in the therapeutic decisions [127].
In breast cancer, mammography scanning method, which relies on X-rays, is both invasive and costly. In contrast, determining CTCs levels through a blood samples collection is simple, non- invasive and not costly. Quantifying CTCs in early-stage breast cancer enables the prediction of early metastatic recurrence in patients. It has been reported that ≥1 CTC/7.5mL in blood samples of patients, whether or not they had received neoadjuvant chemotherapy (NACT), is associated with high risk of relapse and poorer overall survival [128]. Increased levels of circulating tumor cells suggest advancement of metastatic breast cancer. For example, the presence of ≥5 CTCs following initial chemotherapy indicates reduced overall survival, progression-free survival (PFS), and clinical benefit. Assessing CTCs can offer predictive value and help inform decisions on whether to proceed with a second round of chemotherapy or adjust the treatment plan. In Hormone Receptor (HR)- positive, Human Epidermal Growth Factor Receptor 2 (HER2)-negative) metastatic breast cancer, chemotherapy is recommended for patients with CTC counts ≥5 CTCs/7.5 mL, while endocrine therapy is advised for those with <5 CTCs/7.5 mL [129]. In short, it is imperative to note that CTC quantification play a significant role as a prognostic marker to guide clinical decisions.
The enumeration of CTCs through liquid biopsy offers a real-time clinical evaluation, contributing to examining tumor invasiveness after therapy and treatment effectiveness, which helps clinicians adjust therapies accordingly [125]. This real-time evaluation is particularly applicable in in advanced gastric cancer (AGC). In addition, eliminating CTCs may halt metastasis, making them a therapeutic target in prostate cancer. This is proven by Kim, Yoo, Jeong, and Choi (2018) [130], where the improvements in survival rates of the tumor-bearing mice was shown after photodynamic therapy targeting fluorescent protein tagged-CTCs. Reducing the circulation of CTCs in the bloodstream could potentially stop tumors from spreading to other parts of the body. This ultimately helps to prevent traditional therapies such as chemotherapy and radiotherapy, which frequently lead to high death rates.
Overall, measuring circulating tumor cells (CTCs) is crucial for detecting cancer early and predicting prognosis, leading to a more individualized and precise method for managing cancer risk. Recent advancements which complement circulating tumor DNA (ctDNA) and extracellular vesicle (EV) biomarkers with CTCs analysis, have been studied and confirmed to offer a more precise cancer detection [124]. Measurement of cancerous cells counts is beneficial in identifying the underlying of diseases, making it a valuable tool for guiding therapeutic decisions.
Blood disorders
The Complete Blood count (CBC) is a key tool in regular blood tests, checking red blood cells, white blood cells, and platelets [131]. This fast and easy test was conducted by drawing blood and provides a full analysis of a patient's blood makeup. Particularly, the measurement of RBCs, haemoglobin, haematocrit (volume of RBCs relative to the total blood volume) and mean corpuscular volume (MCV) gives crucial diagnostic insights for identification of blood disorders like anemia. Quantifying the blood cells counts is crucial for diagnosing blood disorders, determining their types and severities. This, in turn, assists in directing therapeutic choices for the best possible treatment.
Anemia is a condition marked by a decrease in the amount of haemoglobin or red blood cells. In men, the typical range for RBC count is 5 to 6 million cells/mcL, while for women it is 4 to 5 million cells/mcL [131]. Haemoglobin levels typically range from 14 to 17 gm/dL in adult men, and 12 to 15 gm/dL in adult women. The differences in haemoglobin levels between genders are attributed to hormonal influences, especially testosterone hormone which tends to stimulate higher RBCs production in men [132]. Other factors like altitude, age and race also influence the amount of haemoglobin levels.
MCV is an important measurement that shows the average size and volume of red blood cells, providing diagnostic insight into different forms of anemia [133]. The adjusted reticulocyte counts also indicate bone marrow's RBC production ability in which <2% suggests hypoproliferative anemia, while >2% indicates hyperproliferative anemia, commonly seen in acute blood loss or RBC breakdown (haemolysis). Complemented by the information from the corrected reticulocyte counts <2%, MCV quantification can help classify different types of hypo proliferative anemias [134-136]. There are different types of hypo proliferative anemias, such as microcytic anemia, normocytic anemia, and macrocytic anemia, depending on the levels of MCV.
Upon determining the specific type of anemia by analyzing RBCs, reticulocyte counts, and MCV measurements, healthcare providers can personalize treatment for patients. Intravenous transfusions of RBCs are essential for managing acute blood loss anemia to keep hemoglobin levels at or above 7g/dL [133]. It is imperative to note that increase of red blood cells counts can help manage and treat anemia effectively. MCV quantifications can help predict nutritional deficiencies, guiding the clinician’s decision to prescribe oral or intravenous supplementation. For severe anemias, treatments such as bone marrow transplants may be considered, based on the severity of anemia. Assessing MCV can also lead to additional diagnostic tests or evaluations of specific organs, enabling clinicians to adapt treatments according to the primary cause of anemia.
In essence, it is crucial to comprehend RBC counts and MCV values for diagnosing various forms of anemia and deciding on the best treatment method. These measurements assist in making clinical decisions and enhancing patient outcomes by offering a precise understanding of the type and extent of the blood disorder.
Neuroscience
Aging can lead to the progressive degradation of brain cells, mainly neuron, which are the major contributors to neurological disorders like Alzheimer’s disease. A remarkable feature of Alzheimer’s pathology is the reduction of neuronal densities in the cerebral cortex and hippocampus [137-138]. Typically, Alzheimer's disease is identified by the accumulation of amyloid-β plaques and neurofibrillary tangle, which cause the degeneration of neurons and synapses [139]. This disrupts the connection between brain cells, causing a decline in cognitive function, with main symptoms such as memory loss and difficulty learning.
The human brain consists of various regions, each having unique roles and varied distributions of neurons and non-neuronal cells. The frontal lobe of the brain is where neurons are densely packed in the cerebral cortex. This area is vital for advanced cognitive processes, like reasoning, choosing, and processing sensory data [140]. The hippocampus, also a region with dense neurons, plays a vital part in forming memories. In comparison, non-neuronal cells such as astrocytes, oligodendrocytes, and microglia are evenly spread throughout the brain. These glial cells are extremely important in providing support and protection for the neurons, controlling the chemical surroundings, and participating in immune responses [141].
Chronic neuroinflammation can occur in Alzheimer's disease due to the activation of reactive glial cells in response to amyloid-β plaque and tau tangles. Inflammation can worsen neuronal damage and neurodegeneration [142-143]. Increase in glial cell numbers in the cerebral cortex and subcortical white matter was proven in the scientific finding [137]. Above all, it is important to note that decline in neurons and the increase in glial cells, particularly in the cerebral cortex play a significant role in the progression of Alzheimer’s diseases.
The isotropic fractionator (IF) is a reliable and consistent technique for quantifying brain cells, enabling the measurement of absolute neuron numbers in various brain areas [144]. This method consists of separating fixed brain tissue mechanically to create a consistent mix of individual cell nuclei, which are then counted to determine the total number of cells. Following this, immunocytochemistry is carried out to differentiate between neuronal and non-neuronal cells, such as endothelial and glial cells. By quantifying the absolute neurons and non-neuron cells in the brain of the patients, IF can assess the severity of neuronal loss and detect changes in cells number, assisting in the early detection of Alzheimer’s disease.
Stem cell transplantation is recognized for its ability to transform into specialized nerve cells and enhance neuro-regeneration, as indicated by Schneider et al. (2020) [139]. Different kinds of stem cells such as neural stem cells (NSCs), mesenchymal stromal cells (MSCs), and induced pluripotent stem cells (iPSCs) have been studied for potential treatment of neurodegenerative diseases [145]. NSCs are seen as perfect choices for neurodegenerative illnesses due to their ability to develop into various types of neuronal cells, including neurons, microglia, oligodendrocytes, and astrocytes [145- 146]. NSCs can come from fetal tissues, neonatal tissues (post-mortem), adult brain tissues (specifically from the subventricular zone and dentate gyrus), iPCs, and ESCs [146]. Through NSCs transplantation, cholinergic neurons can be replaced and regenerated, restoring connections between brain neurons and synapses with neighbouring neurons [147].
Transplantation of NSCs derived from human foetal brain into the hippocampus has shown significant enhancements in cognition, primarily in improving synaptogenesis, where the new neurons formed integrate into existing neuronal network. This has been demonstrated in two transgenic murine models of Alzheimer’s disease [148]. Human neural stem cells have been shown to differentiate into NSCs, immature neurons, and glia cells, promoting an increase in endogenous synaptic density [149]. The study confirmed the safety and efficacy of human NSC transplantation in treating Alzheimer’s disease models, suggesting a potential therapeutic solution in clinical settings, as mice share similar physiological traits with humans. Recent research by Angela et al. showed effective human foetal NSC transplantation in a phase 1 clinical trial involving 12 patients with progressive multiple sclerosis, a chronic neurologic disorder [150]. The study showed increased levels of anti-inflammatory and neuroprotective substances in the cerebrospinal fluid of humans, decreased brain shrinkage, and no safety issues. This further validates NSCs transplantation as a potential neuro-regenerative therapy for treating Alzheimer’s disease.
Additional types of stem cells, like IPSCs and ESCs, are capable of producing NSCs cells as well, but tumor susceptibility remains a major concern [146]. Mesenchymal stem cells (MSCs), on the other hand, can differentiate into neurons when exposed to certain growth factors, and are less likely to transform into carcinogenic cells. As a result, they are less prone to immune rejection [151]. Recent research has focused on Alzheimer’s disease treatment using MSCs, due to their ability to multiply and their strong anti-inflammatory abilities [152]. MSCs are known to decrease levels of amyloid-β, stimulate natural microglia, and promote neurogenesis by releasing growth factors.
To recap, it is crucial to comprehend how neurons are spread out in important areas of the brain like the hippocampus and cerebral cortex to reveal the reason for cognitive deterioration. Neuronal loss is the main hallmark of Alzheimer’s disease. Early quantification of neuronal cell loss in the brain serves as a valuable diagnostic tool for assessing the severity of Alzheimer’s disease and can act as a prognostic measure for early intervention. Transplantation of neuron stem cells (NSCs) offers the potential to reverse Alzheimer’s disease by regenerating lost cholinergic neurons and thereby supporting synaptogenesis, eventually helping to restore the cognitive function. All in all, increasing neuronal cells is a promising therapeutic approach for addressing neurodegenerative diseases.
To finish up, by accurately counting different cell types, researchers can gain valuable insights into disease progression and treatment efficacy. Precise cell counts help in understanding an individual’s physiological baseline and can highlight deviations caused by diseases, genetic mutations, or environmental factors. This quantification not only aids in understanding the biological underpinnings of various conditions but also helps in therapeutic interventions. This approach becomes particularly relevant in therapies like immunotherapy, chemotherapy, and regenerative medicine. In the long run, the integration of cell count data into research protocols significantly influences the success rates of new therapies and contributes to more effective clinical outcomes.
Conclusion
Taking everything into account, the complex structure of the human body, comprising roughly 30 to 40 trillion cells, showcases the vast range of cellular functions. Understanding the range of cell types is essential for human biology, but has been difficult to achieve. Previous efforts to quantify human cells often faced limitations due to oversimplified assumptions and incomplete methodologies. Initial predictions were based on broad assumptions that did not have the accuracy necessary to encompass the wide variety of cell types and their differences among people. The lack of advanced tools for precise cell counting in different tissues and organs caused the oversimplifications. Hence, earlier studies provided rough approximations rather than detailed comprehension of cellular composition.
A comprehensive understanding of human cell count estimation is crucial, as it forms the basis for exploring how these cells interact and contribute to overall health, as well as the pathological processes underlying various diseases. Additionally, the consequences of this estimation reach much farther than just numerical data. They provide important guidance for important research in areas like oncology, hematology, and neuroscience, helping researchers understand the intricacies of human health and disease mechanisms. An improved comprehension of cellular makeup boosts our capacity to create precise treatments and new therapeutic approaches, ultimately enhancing patient results.
This information is crucial for the progress of medical research and clinical procedures. As methods progress, upcoming studies are expected to provide further detailed understandings of the cellular composition of the human body. By improving our ability to accurately determine the numbers and types of cells, we can anticipate discovering fresh insights into biological interactions and their potential effects on health and disease. This continuous investigation will not just enhance our knowledge of the human body but also open up new possibilities in medical science.
Conflict of interest
The authors declare that there are no conflicts of interest.